Abstract
Cardiomyopathy encompasses a diverse yet often overlapping spectrum of pathologies and phenotypes. This chapter reviews and discusses the clinical applications of the cardiac imaging modalities to the assessment, diagnosis, and management of cardiomyopathy. Cardiac imaging has a fundamental and established role in providing accurate diagnosis and enhancing identification of those at increased risk of sudden cardiac death, as well as monitoring and guiding therapeutic interventions. While echocardiography remains the cornerstone for initial detection and follow-up of patients with suspected or confirmed cardiomyopathy, other imaging modalities, especially CMR, have gained a central role in the assessment of cardiomyopathy. In this chapter, the contribution of imaging to each diagnosis is discussed separately.
Keywords
Cardiomyopathy, Imaging, Echocardiography, CMR.
13.1
Introduction
13.1.1
Definition and spectrum of disease
The term cardiomyopathy refers to a diverse range of diseases of the heart muscle associated with mechanical and/or electrical dysfunction [ ]. These conditions are of particular importance due to their propensity to cause significant morbidity and mortality, frequently through heart failure and arrhythmia. The myocardial disease may be primary or a secondary consequence of a systemic condition. The classification of cardiomyopathies has evolved since the World Health Organisation’s description of idiopathic cardiomyopathies [ ], largely with the evolution of understanding of these conditions, but this remains somewhat contentious. There is increasing recognition and identification of the genetic basis or contribution to many of the cardiomyopathies, but many questions remain. The most recent classification of the European Society of Cardiology (ESC) is grounded in ventricular morphology and function [ ], in contrast to the American Heart Association (AHA) and American College of Cardiology (ACC), whose classification groups the cardiomyopathies into primary cardiomyopathies, which are subdivided depending on whether they are genetic, acquired or mixed, and secondary cardiomyopathies [ ]. All classifications have some limitations resulting from the diverse and heterogeneous yet overlapping nature of the cardiomyopathies.
13.1.2
Role and challenges of imaging
Much of the classification of the cardiomyopathies relies on the phenotypic description of the structural and functional changes identified by imaging. There is growing recognition of the phenotypic and genetic overlap amongst cardiomyopathies [ ]. As a result, there are a number of challenges in making an accurate diagnosis. Cardiac imaging has become central to the investigation of heart failure aetiology, discrimination between the differential causes of LV hypertrophy, and in informing risk stratification.
The strengths, limitations, and utility of specific techniques are briefly reviewed and then discussed in greater depth with respect to specific conditions. All the imaging techniques have seen considerable advances in their capability due to development of both hardware and software. The technological developments in the cardiac imaging techniques are covered in detail in Part One of this book. Table 13.1 provides a summary comparison of the non-invasive imaging modalities available for the assessment of non-ischaemic cardiomyopathy.
Echo | CT | CMR | PET | SPECT | |
---|---|---|---|---|---|
Scan duration | 20–30 min | 10 min | 30–45 min | 2 h | 1½–2 h |
Contraindications | None | Renal failure Pregnancy | MRI-incompatible implants and devices (relative) Pregnancy during first trimester (precautionary) | Pregnancy | Pregnancy |
Limitations | Operator dependent. Acoustic window Imaging of apical segments and RV | Radiation Currently not suited for detection of fibrosis, perfusion and wall motion Blood flow cannot be assessed | Availability Lower temporal resolution than echo | Availability Low spatial resolution | Availability Low spatial resolution |
Risks | None | Radiation Renal failure Allergy | NSF (with some types of gadolinium-based contrast if severe renal failure) | Radiation Allergy (rare) | Radiation Allergy (rare) |
Radiation | None | 1–10 mSv (depending on scanner and protocol) 1–2 mSv calcium score only | None | 14 mSv (F-18 FDG) | 41 mSv (Thallium stress/rest) 9 mSv (Sestamibi) |
LV/RV function and volumes | ++ 3D echo | + | +++ | – | ++ |
Mass quantification | + (localised hypertrophy can be missed) | + | +++ | – | – |
Oedema | – (non-specific findings such as wall thickening) | – | +++ (STIR sequences) | +++ FDG (uptake) | + (non-specific; areas of reduced perfusion) |
Fibrosis | – | + | +++ | + | – |
While CT has flourished of recent for the assessment of coronary artery disease, perhaps the greatest developments with respect to the imaging of cardiomyopathies have been in cardiovascular magnetic resonance (CMR). With advances in scanner technology and sequences, this technique now offers a “one-stop” shop in the assessment of both ischaemic and non-ischaemic cardiomyopathies.
Specific consideration is given to the imaging of ischaemic heart disease, myocarditis, and contractile function in other chapters in this book.
13.1.3
Echocardiography
Echocardiography remains the initial modality to investigate suspected cardiomyopathy due to its accessibility and availability at relatively low cost. Furthermore, its unrivalled temporal resolution, well-established ability to assess diastolic function, and robust assessment of valvular function, as well as quantitative analysis of features such as strain, have further strengthened its application in the assessment of cardiomyopathy. However, limited ability to clearly discriminate between overlapping phenotypes in cardiomyopathy as well as issues of reproducibility can reduce the power of this technique when applied to myocardial disease. Notably, visualisation of the LV apex and of the right ventricle can be limited on echocardiography and, consequently, some pathologies, such as apical hypertrophic cardiomyopathy, may not be identified. If apical HCM is a possibility, the use of a contrast agent can aid identification of this sometimes subtle pathology (see Chapter 2 ).
In most patients, optimal endocardial definition allows the echocardiographic assessment of LV function. In the minority of subjects where image quality is suboptimal, it is recommended to use contrast echocardiography for LV opacification [ ]. The adjunctive use of contrast echocardiography for enhanced endocardial border definition and more accurate measurement of LV ejection fraction is well described [ , ]. The accuracy of contrast echocardiography remains inferior, even if close, to 3D echocardiography in patients with adequate imaging windows.
With the use of advanced echo techniques such as tissue Doppler imaging (TDI) and speckle-tracking echocardiography (STE), particularly in combination with sophisticated analysis software, regional myocardial function can be accurately assessed by echocardiography [ ]. Although parameters such as velocity, strain, and strain rate (the temporal derivative of strain) obtained by these techniques can offer insight into local myocardial deformation, such deformation imaging is unable to discriminate between active and passive myocardial deformation. TDI measures the velocity of myocardial motion, and measurements at the mitral annulus are validated and widely employed to assess both systolic and diastolic left ventricular function [ ]. This technique has been applied to the assessment of cardiomyopathy with good effect, notably in HCM, as described later in the chapter [ ]. Unlike TDI, STE is largely angle-independent and exploits the speckles that are apparent in grayscale B-mode 2D images. It is an offline technique, and again unlike TDI, can measure motion in any direction relative to the probe. Consequently, both the circumferential and radial components of myocardial deformation can be interrogated. 3D speckle tracking is an even more superior technique, often able to incorporate the entire left ventricle in a single scan volume, allowing true interrogation in any direction and also reduced acquisition time. As with 2D speckle tracking imaging, robust data requires good image quality. For both TDI and STE, measurement of longitudinal deformation is more robust than that of radial deformation.
13.1.4
Cardiovascular magnetic resonance
CMR provides a comprehensive assessment of both ischaemic and non-ischaemic cardiomyopathies. In a single scan, typically lasting around 45 min, detailed information can be obtained with regard to cardiovascular anatomy and cardiac function, blood flow, inducible ischaemia due to epicardial coronary disease or microvascular dysfunction, and tissue characterisation. In the assessment of cardiomyopathy, tissue characterisation by CMR, both using native and extrinsic contrast, is particularly powerful. CMR is also not limited by patient echogenicity, and allows imaging in any plane with excellent delineation of the blood–myocardium interface. Despite these advantages, the quality of CMR images can be markedly reduced by the presence of arrhythmia which can interfere with ECG gating and also by patient difficulty performing breath-holds. A small number of patients are unable to tolerate CMR due to severe claustrophobia, but with experienced staff, adaptations to patient positioning, aids such as prism glasses and reassurance, this is rare. An even smaller number of patients are physically too large to comfortably fit in the bore of a standard clinical scanner. Severe renal impairment carries a potential risk of nephrogenic systemic fibrosis following administration of gadolinium-based agents [ ], but with appropriate consent, this need not be a bar to contrast use providing the indication is robust such that the benefit of the scan outweighs the potential risk. There are also a number of patients who are unable to undergo CMR due to the presence of metallic implants or devices which are not CMR-safe. Given that increasing numbers of patients with cardiomyopathy who require serial imaging are having device implantation, it is of benefit that CMR conditional pacemaker and ICD devices are increasingly available and employed. In addition, there are guidelines and evidence to support safe MR imaging in patients with conventional devices who meet the criteria and where appropriate experience and precautions exist [ , ].
Diagnosis of cardiomyopathy may require the integration of numerous pieces of information and investigations, but CMR can often be definitive in a single test. For example, increased LV wall thickness may be the consequence of hypertension, aortic stenosis, hypertrophic cardiomyopathy, cardiac amyloidosis, sarcoidosis, Anderson–Fabry disease, aortic coarctation, and athletic conditioning, in addition to numerous other substrates. CMR assessment of LV hypertrophy can allow accurate discrimination between these various causes of a hypertrophic phenotype ( Table 13.2 ). It is established that the assessment of LV mass is best done by CMR [ ]. The following briefly summarises the techniques and sequences commonly employed in CMR in the assessment of cardiomyopathy. Greater detail on technological developments in this technique can be found in Chapter 6 .
Abnormal loading |
– Hypertension |
– Aortic stenosis |
– Aortic coarctation |
Hypertrophic cardiomyopathy (predominantly due to sarcomeric protein gene mutation) |
Amyloidosis (Familial ATTR, Wild type TTR (senile), AL amyloidosis) |
Sarcoidosis |
Lyosomal storage disease (e.g. Anderson–Fabry) |
Glycogen storage diseases (Danon, Pompe) |
Friedrich’s ataxia |
Drug-induced (Tacrolimus, hydroxychloroquine, steroids) |
Intense athletic conditioning |
Noonan syndrome/LEOPARD syndrome/Costello syndrome |
Mitochondrial disease |
CMR is the gold standard for assessment of ventricular volumes given its accuracy and reproducibility [ ]. Of all the techniques, it is currently the most able to deal with the variable anatomy of the right ventricle and generate robust quantitative assessment of volume and function. The routine practice of quantification of RV volumes and function enhances the accuracy of this.
Differences in LV volumes acquired by different modalities are widely reported such that it is important to be aware that measures obtained by different modalities are not interchangeable. This will consequently impact on eligibility where EF is used as a criteria and the majority of existing literature is based on echocardiographic measurement of EF. Previous studies have highlighted this point, although few have quantified the impact of this in specific relevant populations, either in terms of cost or outcome. The incorporation of CMR assessment in major HF trials is important.
The protocol for CMR assessment of cardiomyopathy is a relatively standard protocol, although this may be modified based on the clinical question. Typically dark-blood anatomical images are acquired using a multi-slice single shot spin-echo sequence (Half-Fourier Acquisition of Single-Shot Turbo Spin Echo, HASTE) in trans-axial, coronal, and sagittal planes. Bright-blood images may be acquired instead or as well using steady-state free precession (SSFP) imaging. Cine-CMR images are then acquired using cine-SSFP imaging to provide functional information.
Tissue characterisation exploits either intrinsic tissue properties (non-contrast tissue characterisation) or the interaction of extrinsic contrast agents (specifically gadolinium-based contrast) with tissues. Specific sequences have been developed to enable the identification of pathological tissue.
Short-tau inversion recovery (STIR) sequences are T2-weighted sequences with increased sensitivity to myocardial fluid content. Signal from flowing blood and fat is suppressed and the physical properties of the sequence are designed to yield high signal in regions of oedematous tissue. Consequently, regions of acute myocyte swelling and interstitial oedema can be identified, although as such are relatively non-specific. The technique can be limited by interference from high signal in regions of low velocity blood flow, notably at the LV apex and in regions of prominent trabeculation, by variation in proximity to the surface coil, low signal-to-noise ratio and the standard sources of artefact. Additionally, interpretation is usually subjective, often informed by comparison with late gadolinium enhancement. The subjectivity and limitation in detecting more global oedema can be improved by comparing myocardial signal intensity with skeletal muscle as a reference or better still by using T2 mapping techniques. Any of the mapping techniques simply creates a spatial representation of a particular signal, be it flow velocity, T2 value, or T1 values.
Recent CMR literature has been dominated by the numerous sequences and techniques which aim to identify diffuse fibrosis through T1 mapping and extracellular volume quantification. T1 parameters can be interrogated either with or without utilisation of a gadolinium-based contrast. The current gold standard technique for non-invasive determination of the ECV is the equilibrium contrast method [ , ]. There is now much data using these techniques, yet they are not universally employed clinically for a variety of reasons. There is currently a wide heterogeneity of protocols and sequences with limited data across vendors [ ]. White et al. [ ] show that there is a systematic overestimation of ECV in high ECV diseases with bolus only protocols for ECV quantification compared to measurement obtained by the equilibrium contrast method and histology.
T2* sequences exploit the more rapid destruction of signal by iron after radiofrequency excitation to identify myocardial iron loading and are uniquely powerful in this respect.
Gadolinium-based contrast agents are extracellular and can be utilised in CMR in a number of ways to generate additional information. As well as being administered for MR angiography, they can be used in combination with vasodilator stress to assess first pass myocardial perfusion, identifying inducible myocardial ischemia. In the minutes after administration, the presence of gadolinium in the blood pool can identify filling defects due to thrombus, and there may also be discernible changes in myocardial signal intensity in the presence of myocardial pathology. Gadolinium accumulates where there is expansion of the interstitial space and after at least 5 min is allowed for this to occur, LGE imaging can identify areas of myocardial infarction, fibrosis, oedema, or infiltration. The pattern of enhancement can inform both diagnosis and prognosis. This may be one of the most useful tools in informing the aetiology of heart failure in increased LV wall thickness. In the conditions studied so far, the presence of LGE confers an adverse prognosis compared to its absence [ , ].
Tagging sequences superimpose a grid or similar pattern with radiofrequency excitation and allow visualisation of tag deformation. This not only provides readily interpretable subjective information, but can be analysed in a number of software packages to provide quantitative analysis of deformation parameters.
13.1.5
Computed tomography
Multi-detector CT (MDCT) is emerging as a valuable adjunct in the assessment of cardiomyopathy to rule out coronary artery disease in place of invasive angiography, customarily undertaken as part of the assessment of newly diagnosed heart failure. Probably the greatest advance in CT with consequent increase in the utilisation of this technique is in relation to radiation dose reduction (see Chapter 5 ). Acquisition can take under one minute and is not generally limited by the presence of devices or implants. The strength of CT coronary angiography is in its negative predictive value and consequently should be utilised in those with no more than a low-intermediate probability of significant epicardial coronary artery disease. CT perfusion has recently been introduced as a first-pass contrast technique to assess myocardial ischemia. Although first results with this novel technique are promising, some practical issues still limit the widespread acceptance of this technique, including low myocardial contrast, beam-hardening artefacts, and considerable radiation exposure. Delayed contrast enhancement protocols for demarcating fibrotic scar have also been tested in some experimental settings but are not routine yet, and may be more difficult in diffuse patterns of myocardial fibrosis. The risk of nephropathy associated with the iodine-based contrast agents used for CT can also be limiting. Nonetheless, with its very high isotropic spatial resolution which for the newest devices is lower than 0.3 mm, CT may be a valuable alternative to CMR for certain cardiomyopathies, whenever CMR is contraindicated. Evidence of ventricular dilatation, increased wall thickness, or subclinical myocardial infarction may be noted incidentally at cardiac CT. Cardiac CT with extended exposure throughout the heart cycle allows for assessment of regional and global LV function, myocardial motion and thickening, and measurements of RV parameters, albeit at a higher radiation exposure than conventional CT coronary angiography.
13.1.6
Nuclear imaging
Radionuclide imaging is generally not the first-line modality for the assessment of cardiomyopathies, unless when imaging is considered for excluding ischemic cardiomyopathy. The spatial resolution of current positron emission tomography (PET) or single-photon emission computed tomography (SPECT) is in the range of 4–5 and 8–10 mm, respectively, and therefore these techniques are not suitable for detecting structurally abnormal myocardium (see Chapters 3 and 4 ). However, radionuclide techniques are notable for their high molecular sensitivity which allows for the detection of radiotracer in nanomolar concentrations. Therefore, these techniques are employed to address specific pathobiological and functional changes in the myocardium that escape anatomical imaging modalities.
18 F-fluorodeoxyglucose and 67 Gallium are avidly taken up by activated macrophages and therefore allow detection of inflammatory infiltrates, notably in cardiac sarcoidosis. Myocardial perfusion PET with 82 rubidium, 13 N-NH 3 or 15 O-H 2 O allows quantification of myocardial blood flow in mL/min/g, and thereby is considered the gold standard technique to detect microvascular dysfunction. Several cardiomyopathies have been shown to coexist with variable severities of impaired microvascular vasoreactivity, and the most severe forms are generally associated with a worse cardiovascular prognosis. Finally, myocardial sympathetic innervation can be interrogated with radiolabeled structural analogues of norepinephrine (e.g. 131 I-meta-iodo-benzylguanidine (MIBG) or 11 C-meta-hydroxyephedrine (MHED)). A number of cardiomyopathies (in particular hypertrophic, dilated, and arrhythmogenic right ventricular cardiomyopathy (ARVC)) may be associated with increased sympathetic activity, which in turn has been linked with a higher likelihood of unfavourable ventricular remodelling, progression to heart failure, and a higher risk of arrhythmic events and cardiovascular mortality. However, although radionuclide imaging has enhanced our pathophysiologic understanding of many cardiomyopathies, its use is currently limited to experimental investigations rather than clinical routine. Additional issues are limited availability of radiolabelled compounds, radiation exposure, and costs.
Finally, radionuclide ventriculography (RNV) using either first-pass or blood-pool imaging can be used to quantify left- and/or right ventricular ejection fraction and regional contraction patterns and mechanical synchronicity. With the widespread use of echocardiography and CMR, RNV has lost importance. However, in patients with poor acoustic windows and contraindications to CMR, RNV represents a useful alternative imaging test to measure and quantify ejection fraction.
13.2
Dilated cardiomyopathy
13.2.1
Diagnosis
Dilated cardiomyopathy is defined by left ventricular dilatation and systolic dysfunction in the absence of abnormal loading conditions (namely hypertension or valvular heart disease) or ischaemic heart disease of sufficient severity to explain the observed ventricular derangement. Dilatation and impairment of the right ventricle may also be present, but are not required for the diagnosis. The atria are also commonly dilated.
A dilated cardiomyopathy phenotype ( Figure 13.1a and b ) may result from a wide variety of causes (including familial, infectious, toxin mediated, metabolic, inflammatory, infiltrative, connective tissue and neuromuscular causes, as well as pregnancy and arrhythmia related). In up to 50% of cases, no aetiology is identified and the disease is termed idiopathic. With increasing identification of genetic substrates [ ], now thought to contribute to at least 25% of dilated cardiomyopathies, this label is becoming less prevalent. However, the interplay between genetic and acquired factors requires much further elucidation [ ].
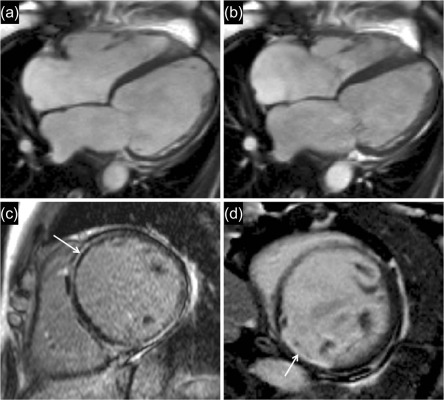
Demonstration of LV dilatation (end diastolic diameter > 117% predicted value normalised for age, gender, and body surface area) and systolic dysfunction (LVEF < 45% and/or fractional shortening < 25%) by echocardiography continues to be the mainstay of diagnosis [ ]. Typically, there is global hypokinesia. However, regional variation in wall stress or coexisting myocardial infarction may give rise to regional wall motion abnormalities.
An important role of non-invasive imaging in dilated cardiomyopathy relates to exclusion of an ischemic origin. Several techniques have the ability to detect or exclude with high accuracy underlying coronary artery disease, although in many centres, such patients will be submitted to invasive angiography with the ability to perform simultaneously right heart catheterization and measuring pulmonary pressures. CT coronary angiography, however, represents a valuable alternative to invasive angiography in patients with low-to-intermediate likelihood of coronary artery disease [ , ]. However, these patients pose technical challenges to conventional CT coronary angiography: They tend to have higher heart rates, and betablockade may be problematic, leading to lower image quality and more motion artefacts. Furthermore, circulation times may be longer, thereby increasing the time for the contrast bolus to arrive in the coronary arteries. This may result in lower signal-to-noise ratios and more difficult scan timing. Furthermore, larger contrast amounts may be required to obtain high image quality with potentially higher nephrotoxicity. A calcium scan may be an alternative, contrast-medium free approach to exclude ischemic CMP. Although non-calcified coronary stenoses are frequently encountered, the probability of extensive CAD (as would be expected in dilated CMP caused by diffuse myocardial ischemia) is extremely rare [ ]. Because the specificty of the calcium scan is low, the calcium scan is most effective in patients with a relatively low pre-test probability.
Radionulide myocardial perfusion imaging has demonstrated a high sensitivity and negative predictive value to exclude an ischemic origin in patients with dilated cardiomyopathy [ ]. However, specificity is somewhat limited by false positives occurring in the presence of attenuation artefacts or microvascular dysfunction.
Over recent years, the application of CMR to the assessment of DCM has expanded notably due to the accurate and reproducible assessment of cardiac morphology and function and the capability for non-invasive identification of myocardial fibrosis and inflammation, as well as myocardial infarction. The majority of patients with DCM have no LGE. However, midwall myocardial fibrosis ( Figure 13.1c ) has been found to be present in approximately one-third of DCM patients as identified by LGE and this has been well validated against histological specimens. This midwall LGE is in contrast to the subendocardial or transmural pattern of LGE corresponding to myocardial infarction ( Figure 13.1d ).
13.2.2
Prognostic imaging markers
Prognostic markers in DCM include clinical factors, biochemical markers, electrophysiological parameters, and haemodynamic parameters. There is now considerable data supporting imaging parameters as powerful prognostic indicators. It has been apparent for some time that echocardiographic assessment of LV remodelling can provide prognostic information in DCM [ ]. LV ejection fraction consistently appears as an independent predictor of outcome in the literature [ ]. Several other parameters obtained by echocardiography have also been found to confer prognostic information in DCM. These include evidence of RV involvement as assessed by TAPSE [ ], the presence of a restrictive filling pattern [ , ] and the severity of mitral regurgitation [ ]. Venturi et al. [ ] found the effective orifice area of functional mitral regurgitation to be a better predictor of NHYA class and mortality than LV EF.
Both strain and strain rate are reported to be reduced in DCM, again associated with poorer outcome [ ].
Assomull et al. [ ] have shown late gadolinium enhancement to be an important predictor of adverse outcome. In a cohort of 101 consecutive DCM patients followed up prospectively for 658 ± 355 days for events; 35% had midwall fibrosis as evident on CMR by LGE. This was associated with a higher rate of the predefined primary combined end point of all-cause death and hospitalization for a cardiovascular event (hazard ratio 3.4, p = 0.01). Midwall fibrosis remained predictive of SCD/VT after correction for baseline differences in left ventricular ejection fraction between the group with and the group without LGE. Wu et al. [ ] and Lehrke et al. [ ] similarly found the presence of LGE in non-ischaemic cardiomyopathy to be associated with marked increase in cardiac events.
Left atrial size has long been considered a barometer of the cardiovascular system, with both maximum and minimum size carrying prognostic information [ ]. Left atrial volume has now been established as a prognostic marker across a number of cardiovascular diseases including DCM [ ] and HCM [ ]. In a cohort of 483 consecutive DCM patients, Gulati et al. [ ] found that an indexed left atrial volume of more than 72 mL/m 2 carried a threefold elevated risk of death or transplantation (HR 3.00; 95% CI 1.92–4.70; p < 0.001).
While these data highlight the potential value of these parameters in risk stratification, the way these inform patient management requires further consideration and elucidation.
Microvascular dysfunction occurs in dilated cardiomyopathy in the absence of epicardial coronary artery disease and is an important risk factor. Neglia and colleagues reported reduced hyperemic blood flow in patients with dilated cardiomyopathy and an ejection fraction of 34% on average [ ]. A low hyperemic blood flow emerged as a strong and independent predictor for cardiac mortality or progression/development of heart failure. A hyperemic blood flow < 1.36 mL/min/g increased the risk of death or heart failure by a factor of 3.5.
Abnormal cardiac sympathetic activity is common in dilated cardiomyopathy and plays an important role in the LV remodelling and progression of heart failure, including its complications of arrhythmia and sudden death. Cardiac sympathetic activity can be evaluated by its uptake of radiolabeled catecholamine analogues like MIBG with SPECT. In dilated cardiomyopathy, low MIBG uptake (indicating increased sympathetic innervation) is one of the strongest predictors of outcome (even stronger than EF), and provided independent prognostic information [ , ]. These results have recently been replicated in large multicentric registries [ , ] consisting of a mixed population of nonischemic and ischemic cardiomyoapthies confirming the independent prognostic value of MIBG SPECT of ejection fraction, NYHA functional status, and biomarkers (e.g. pro-BNP).
13.2.3
Non-invasive imaging as a gatekeeper for coronary angiography
Current US guidelines prioritise the identification of coronary anatomy either by invasive angiography or CTCA in the assessment of heart failure and the exclusion of coronary artery disease as a substrate for LV dysfunction is ubiquitous. Given significant reduction in radiation dose, increased availability and advances in image quality, recent years have seen a marked increase in the utilisation of CTCA for this, notably where the likelihood of coronary artery disease is thought to be low given the excellent negative predictive power of this investigation. The identification or exclusion of ischaemic heart disease and flow limiting coronary artery disease is also an area where CMR has much to offer. The ability of CMR to identify myocardial infarction and inducible ischemia in a single test (by LGE and stress perfusion respectively) raises the possibility of using this as a gatekeeper to coronary angiography as proposed by Assomull et al. [ ].
It will take either strong evidence or other pressures, perhaps financial to produce a shift from the traditional assessment of invasive coronary imaging in patients with newly diagnosed heart failure. A persistent challenge in functional assessment by DSE, nuclear or perfusion CMR is with false negative and false positive studies. In selecting the appropriate test, it is important to evaluate the pre-test likelihood of the patient having coronary artery disease. The role of CT is for ruling out coronary artery disease given its high negative predictive value, although the development of CT assessment of FFR may see this evolve.
13.2.4
Chemotherapy-related cardiomyopathy
A number of chemotherapeutic regimes are well recognised to carry cardiotoxic side effects. Amongst these, the anthracyclines (doxorubicin, daunorubicin, and epirubicin) and the HER2 receptor modulator Trastuzumab carry significant risk meriting cardiac assessment prior to, during and following therapy. Cardiotoxicity is typically dose dependent and is also influenced by a number of other factors. The prudent application of imaging before, during and after chemotherapy can enable safer use of potentially cardiotoxic agents by identifying LV dysfunction early when chances of reversibility are greater. A recent international consensus statement details recommended multi-modality imaging strategies for surveillance and detection of cardiotoxicity [ ]. In this document, cancer therapeutics-related cardiac dysfunction (CTRCD) is defined as a decrease in LVEF of more than 10% points to a value of below 53% (the lower limit of the normal reference range by 2-D echocardiography). Confirmation of this change on repeat study 2–3 weeks after the initial detection of decreased LVEF is recommended. LVEF is the parameter typically used for assessment of LV systolic function and as with other cardiomyopathies, echocardiography with LV volume quantification and LVEF calculation by the modified biplane Simpson’s technique is at the core of imaging evaluation. The volumetric method for calculating ejection fraction increases detection of CTRCD that due to some agents may be regional rather than global. CMR and MUGA are also robust options for quantification of LV volumes and ejection, although utilisation of the former may be constrained by limited availability and the latter by the incumbent ionising radiation burden. Bountioukos et al. [ ] found that incorporating a wall motion score index obtained by 2-D echocardiography using a 16-segment model increased sensitivity for anthracycline cardiotoxicity, a practice encouraged in the consensus statement. In the same study, Bountioukos et al. found that repetitive assessment of contractile reserve using low-dose dobutamine stress echocardiography did not have incremental value for the early detection of anthracycline cardiotoxicity. Although an asymptomatic reduction in LVEF is the most frequently observed clinical manifestation of cardiotoxicity, LVEF is relatively insensitive to the detection of subclinical myocardial injury. Small changes in LV contractility may not be detected by the 2D echocardiographic assessment of LVEF. Thavendiranathan et al. [ ] assess the temporal variability in LVEF by echocardiography in 56 patients undergoing cancer chemotherapy in whom stable LV systolic function was inferred by stability of global longitudinal strain (GLS) at up to 5 time points. They reported the limit of the 95% confidence interval for longitudinal variability of 2-D LVEF management in the asymptomatic patients to be 9.8% (range 9.0–10.8%). Nonetheless, echocardiography remains the method of choice for serial evaluation of patients requiring potentially cardiotoxic agents. If available, 3D echocardiography is preferable for calculation of LVEF. The newer techniques for interrogating systolic function, such as myocardial strain and strain, have much greater sensitivity for changes in myocardial tissue deformation and in CRTCD, as in other conditions, can enable detection of subclinical disease [ , ]. GLS measured by 2-D speckle tracking echocardiography is considered the optimal parameter of myocardial deformation for the early detection of subclinical LV dysfunction as it is not angle dependent, unlike diffusion tensor imaging (DTI). It is recommended that measurements be compared with baseline and that a greater than 15% reduction from baseline indicates clinically significant abnormality [ ]. If assessment of GLS is not available, using pulse wave DTI to assess peak systolic velocity ( s ′) of the mitral annulus or M-mode echocardiography to assess mitral annular displacement is recommended.
Loss of torsional motion is an even earlier feature of cardiomyopathy in this setting, and may be evident before loss of longitudinal or radial function or significant reduction in ejection fraction [ ]. This can be visualised by echocardiography or CMR and quantified with the use of 2D speckle tracking or myocardial tagging sequences in the respective techniques. Unlike assessment of LV systolic function, assessment of LV diastolic function has not yet been shown to provide prognostic data in this setting but should still be included in the routine echocardiographic assessment of such patients. Additional consideration of CMR or CT is highlighted for the evaluation of primary cardiac tumours or when echocardiographic assessment has been inconclusive in the evaluation of possible constrictive pericarditis.
Some chemotherapeutic agents, for example fluorouracil, bevacizumab, sorafenib, and sunitinib, are recognised to potentially precipitate myocardial ischaemia. Evaluating patients due to receive such agents and who have an intermediate or high pre-test probability for coronary artery disease with stress echocardiography may be of value. Beyond this, the role of dobutamine stress echocardiography in the assessment of patients with CTRCD remains somewhat inconclusive, although using this to assess contractile reserve may be of benefit. Reduction in contractile reserve demonstrated in this manner may allow earlier detection of cardiotoxicity as well as predicting outcome.
13.3
Non-compaction cardiomyopathy
Left ventricular non-compaction (LVNC) is recognised as a distinct cardiomyopathic entity characterised by hypertrabeculation ( Figure 13.2 ) of the left ventricular myocardium resulting from failure or arrested compaction of the myocardium in utero. Consequently the myocardium has a spongy appearance with characteristically excessive trabeculation and deep intra-trabecular recesses [ ]. This structure predisposes to thrombus formation as well as to ventricular arrhythmia. In progressive disease, this may cause significant systolic impairment. Although hypertrabeculation may also be evident in the right ventricle, it is typically the LV apex and lateral wall where the features of LVNC are most commonly seen. The relatively thin noncompacted layers often display hypokinesia. Diagnosis is heavily reliant on advanced imaging with echocardiography or CMR. The superior ability of CMR to clearly visualise the apical myocardium is of potential advantage in assessing this condition, although diagnosis is frequently made by echocardiography. There is discrepancy between echo and CMR criteria for non-compaction cardiomyopathy as published by Jenni et al. [ ] and Petersen et al. [ ], respectively. It is likely that these will be revised in the near future. Diagnosis by echocardiography requires a ratio of non-compacted to compacted myocardium of greater than 2.0 in systole compared to a ratio of > 2.3 at end-diastole by CMR. It is also problematic that features of non-compaction are often seen when there is marked LV dilatation, which can make distinguishing primary non-compaction cardiomyopathy from the appearance of non-compaction due to LV dilatation difficult. Whilst LVNC may represent a previously under-recognised cause of heart failure, it may be that diagnostic features lack sufficient specificity. Kohli et al. [ ] suggest that diagnostic echocardiographic criteria for LVNC may be too sensitive, most notably in Black patients. The use of an appropriate contrast agent is helpful in the identification of ventricular thrombi regardless of technique. Observed to have a largely autosomal dominant inheritance, a genetic basis underlying this condition has been well defined, with mutations defined in three sarcomeric genes [ ].
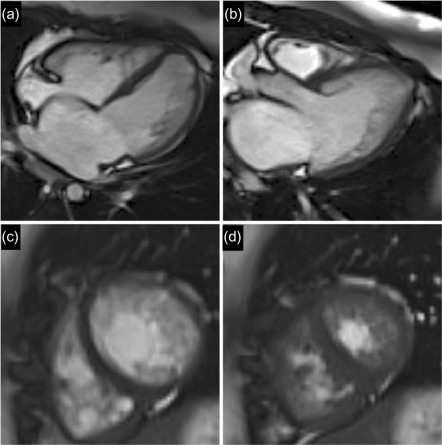
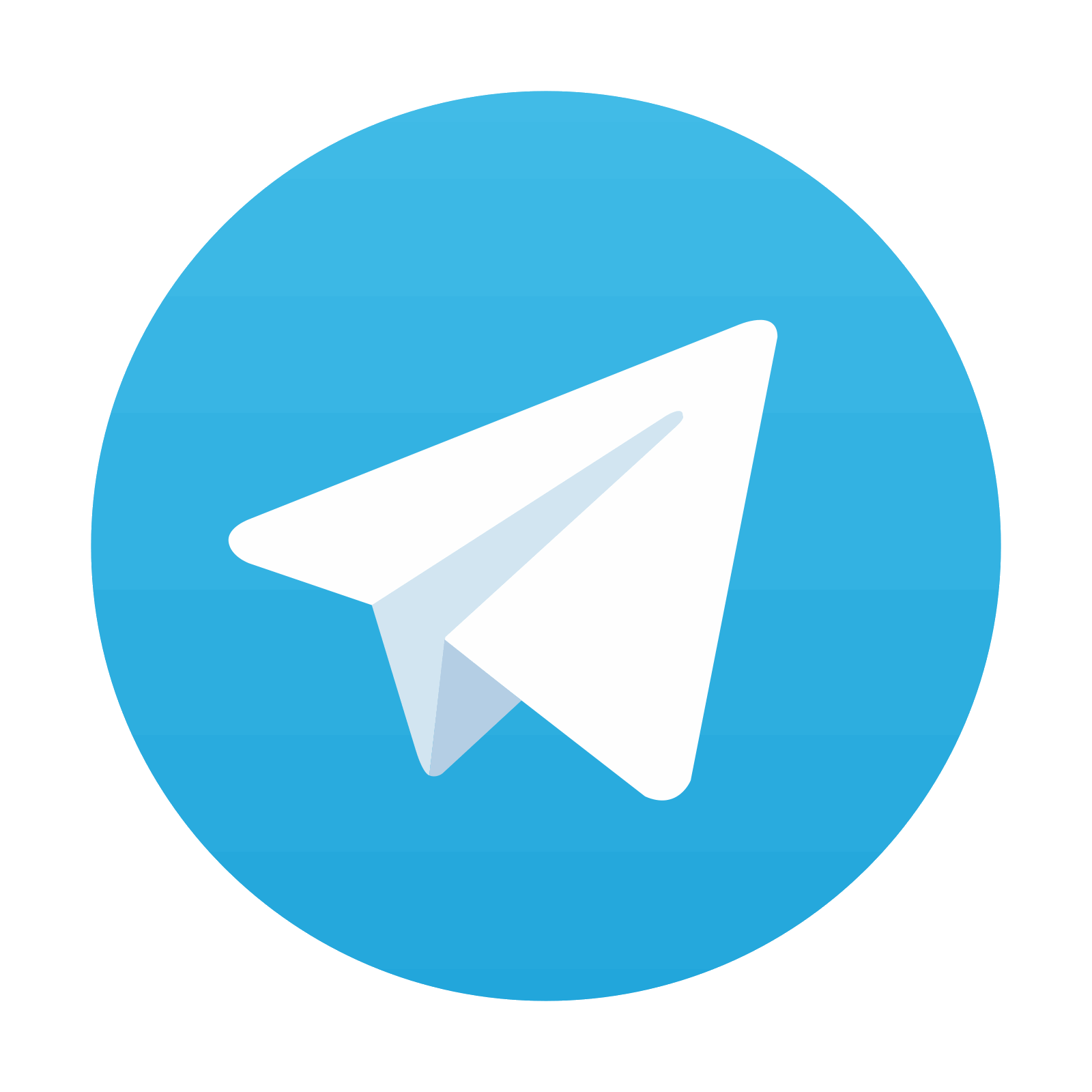
Stay updated, free articles. Join our Telegram channel
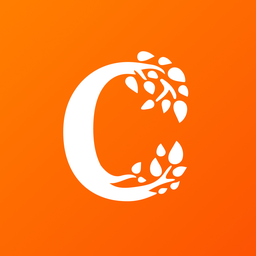
Full access? Get Clinical Tree
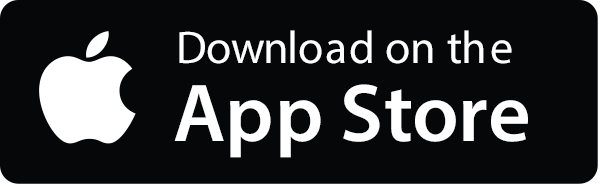
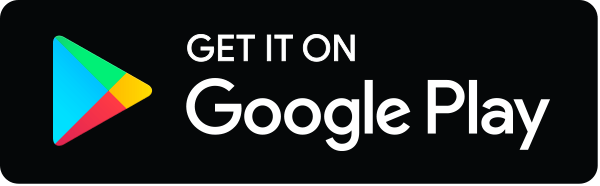
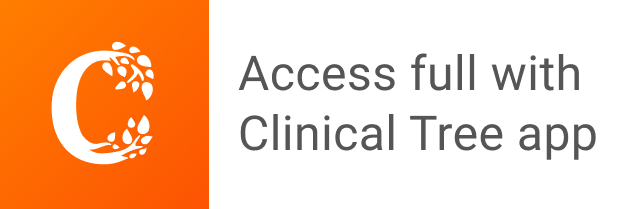