Although death rates in industrialized countries have been consistently falling since the 1980s, atherosclerosis is now raging throughout the developing world. As a consequence the complications of atherosclerosis have become the leading cause of mortality and morbidity worldwide. Fundamentally, atherosclerosis is an inflammatory disease, which affects medium and large arteries from the first decade of life until death. It has a predilection for certain arterial beds, with the carotid artery and aorta being the most common sites of plaque formation. In the carotid circulation, the end result can be an ischemic cerebral insult causing either temporary (transient ischemic attack [TIA]) or permanent (cerebrovascular attack [CVA]) symptoms. If unchecked, aortic atherosclerosis can predispose to dissection, intramural hemorrhage, aneurysm formation and downstream embolus. Importantly, because atherosclerosis is a systemic disease, assessments of the carotid arteries and aorta also provide surrogate information about disease burden and activity in the coronary circulation, providing prognostic information about the risk of myocardial infarction.
Pathobiology of Atherosclerosis
Atherosclerosis is characterized by the gradual accumulation of lipid, inflammatory cells, and connective tissue within the arterial wall. It is a chronic, progressive disease with a very long asymptomatic/subclinical phase. The first abnormality to develop is the fatty streak, caused by the collection of lipid and macrophages in the subendothelial space. Fatty streaks develop primarily in regions of low wall shear stress, which results in endothelial dysfunction and the production of less nitric oxide. The major atherogenic risk factors including smoking, hypertension, and diabetes mellitus also all affect endothelial function, impairing both its barrier function and secretory capacity. Ultimately the blood vessel wall becomes more permeable to blood-derived lipids and inflammatory cells in these regions, encouraging the early stages of atherosclerosis.
Once within the subendothelial space, low-density lipoprotein (LDL) becomes oxidized and attracts monocytes by triggering the release of monocyte chemoattractant protein-1 (MCP-1) from the overlying endothelial cells. Endothelial adhesion molecules, including vascular cell adhesion molecule-1 (VCAM), intercellular adhesion molecule-1, E-selectin, and P-selectin, facilitate the internalization of further monocytes. Once they have escaped the blood pool, monocytes transform into macrophages and bind and internalize oxidative LDL via their scavenger receptors. Eventually, the subendothelial accumulation of modified LDL and macrophage-derived foam cells leads to the formation of the atheromatous lipid core. The thrombogenic components of the lipid core become separated from blood in the lumen by the endothelialized fibrous cap, which consists predominantly of vascular smooth muscle cells (VSMCs) and connective tissue. VSMCs migrate from the arterial media and synthesize the extracellular matrix components of the cap, such as elastin and collagen. The cap also contains variable numbers of inflammatory cells, most importantly macrophages. As the plaque enlarges, the affected artery grows outward (by expansion of the external elastic lamina) so that lumen diameter and therefore blood flow is initially preserved; this is a process known as positive remodeling. As the artery’s wall stress increases with outward remodeling, further expansion eventually becomes impossible and the plaque then encroaches into the lumen. Ultimately this may cause angina by compromising blood flow to the myocardium.
Mature plaques may also become calcified, a process that preferentially affects the intima of the artery and is thought to occur as a healing response to inflammation and cell death within the plaque. Although the early stages of microcalcification are associated with high-risk inflamed atheroma, the latter stages of macrocalcification are by contrast associated with burnt-out stable disease. Very advanced plaques with a large necrotic core and associated hypoxia will also often be perforated by new blood vessels under the influence of angiogenic factors, a process called neovascularization , with similarities to that which occurs within growing tumors. However, these small arteries are structurally fragile and have a tendency to spontaneously hemorrhage, which can destabilize the plaque leading to increased plaque inflammation, rupture, and the precipitation of acute myocardial infarction.
Atherosclerotic plaques may remain quiescent for decades. However, when they initiate clot formation in the vessel lumen, they can very quickly become life threatening. This occurs predominantly as a result of fibrous cap rupture, with consequent exposure of the thrombogenic and tissue factor-rich lipid core to circulating blood. Less commonly, there can be erosion of the endothelial cell layer overlying the fibrous cap, which in the context of an advanced thrombogenic response can also lead to intravascular thrombosis. It is estimated that endothelial erosion accounts for ~30% of myocardial infarctions. Regardless of the mechanism, plaque disruption will lead to varying degrees of local platelet activation and thrombus formation, depending on the characteristics of the plaque and the thrombogenicity of the blood. Importantly, however, the majority of these plaque ruptures do not appear to result in overt clinical events but instead remain subclinical and responsible for abrupt plaque growth.
It has become clear that the cellular and extracellular composition of the plaque is the primary determinant of plaque stability. Lesions with a large lipid core, thin fibrous cap, positive remodeling, angiogenesis, microcalcification, and a preponderance of inflammatory cells compared with VSMCs are at the highest risk of rupture. Inflammatory cells, particularly macrophages, produce metalloproteinase enzymes, which break down matrix proteins, weakening the fibrous cap. In addition, they secrete inflammatory cytokines, in particular interferon γ, which inhibit VSMC proliferation and matrix production. Furthermore, VSMCs in the fibrous cap have a reduced proliferative capacity and a propensity to apoptosis. Consequently, inflammation within the plaques promotes destruction of the fibrous cap, a propensity to plaque rupture, and subsequent thrombosis. However, this is balanced by the action of VSMCs, which nourish and repair the cap, promoting plaque stabilization. This dynamic balance between proinflammatory and healing responses across the vasculature ultimately governs a patient’s risk of myocardial infarction or stroke. These processes are independent of the degree of luminal stenosis. Consequently, positively remodeled and angiographically invisible plaques can rupture to precipitate a fatal clinical event, while many large plaques that obstruct flow and cause angina may be stable and not life threatening. There is therefore an urgent need for imaging techniques that can discriminate “stable” from potentially “unstable” lesions in clinical practice. This chapter will investigate the role that cardiovascular magnetic resonance (CMR) imaging might play in this respect, with a focus on its ability to measure the total plaque burden, to investigate high-risk plaque characteristics, and to determine disease activity with advanced molecular techniques.
Imaging Atherosclerosis
CMR can make use of differences in tissue relaxation times (T1 and T2) and proton density, intrinsic material properties that affect the magnitude of the magnetic resonance (MR) signal, to generate soft-tissue contrast, providing detailed information about atherosclerotic plaque composition. This is readily feasible in the large and relatively immobile carotid arteries and thoracic aorta, with intensive research aimed at transferring these techniques into the more challenging coronary vasculature.
The aim of atherosclerotic plaque imaging is ultimately to identify vulnerable patients at high risk of myocardial infarction or stroke before symptoms and complications develop. Similarly, early identification of disease might allow the implementation of pharmacologic treatment to halt or even reverse the process of plaque progression and possibly prevent rupture (e.g., with statin therapy). Recently, rapid advances in CMR hardware, software, and molecular imaging tracers have seen CMR develop into a modality of considerable utility and versatility for imaging large vessel atheroma. In particular, assessments of atherosclerotic plaque burden offer powerful prediction of adverse cardiovascular events, whereas more advanced techniques aimed at evaluating high-risk plaque characteristics and disease activity hold promise in refining this risk prediction even further. Each of these features will be discussed in detail below.
Assessments of Plaque Burden
Simple measures of the atherosclerotic plaque burden in different vascular beds provide powerful prognostic information, presumably because the more plaques a patient has the more likely one will rupture or erode and cause an event. CMR can effectively image atherosclerotic plaque using high-resolution, black-blood, fast-spin echo sequences. Preparatory pulses null signal in the blood pool and perivascular fat, improving contrast between the plaques and vessel lumen, and surrounding tissue, respectively. The atherosclerotic plaque burden can then be quantified using measurements of plaque thickness in two dimensions and plaque volume in three dimensions. In the aorta these measurements demonstrate a close correlation with transesophageal echocardiography and with increasing numbers of cardiovascular risk factors. Similar approaches have been used to measure plaque thickness and the plaque volume in the carotid arteries, providing measures of atherosclerotic burden that compare favorably with histology and predict major adverse cardiovascular outcomes.
Black-blood CMR also allows the early detection of subclinical atherosclerotic disease. Indeed CMR is particularly attractive for this purpose, given the absence of ionizing radiation, and has already proved useful in several large cohort studies. In asymptomatic subjects enrolled in the Framingham Heart Study (FHS), FHS coronary risk score was strongly associated with asymptomatic aortic atherosclerosis detected by CMR. The prevalence and extent of aortic atherosclerosis increased with age. In a substudy of the Multiethnic Study of Atherosclerosis (MESA), CMR aortic wall thickness increased as a function of age, but males and black participants had the greatest wall thickness. In another study of 102 patients undergoing x-ray coronary angiography, aortic atherosclerotic plaques were detected with a higher frequency in active smokers and in those with high levels of LDL-cholesterol, but the volume and area of aortic plaques correlated most strongly with age and the presence of hypertension. Interestingly, only atherosclerotic plaques located in the thoracic aorta were found to be associated with coronary artery disease (CAD). Taken together, these studies confirm the strong correlation between the presence of cardiovascular risk factors and the incidence of aortic atherosclerosis. It should be noted, however, that there are racial and population differences in the response to individual risk factors, which presumably have a genetic basis.
Another benefit readily appreciated by pharmaceutical companies and others wishing to study the effects of drugs on plaque progression (and regression) is the low coefficient of variability of CMR plaque volume measurements. This translates directly into the requirement for lower patient numbers for studies investigating the impact of pharmaceutical agents on plaque volume, because any true drug effect will not be swamped by noise within the measuring technique. This was illustrated in two separate studies where high-dose statin regimens were shown to be superior to low-dose regimens in terms of atheroma burden reduction, an effect that could be demonstrated with only 20 patients per group.
Subsequently, in the first double-blind, multicentric, dal-PLAQUE (safety and efficacy of dalcetrapib on atherosclerotic disease using novel noninvasive multimodality imaging) study, 130 patients were randomly assigned to placebo ( N = 66) or 24 months treatment with the cholesterylester transfer protein (CETP) inhibitor dalcetrapib ( N = 64). In patients receiving dalcetrapib, the absolute change from baseline relative to placebo in total vessel area was 4.01 mm 2 (90% CI, 7.23 to 0.80; nominal P = .04) over 24 months.
Plaque Characteristics
Plaques at risk of rupture have certain pathological characteristics, including inflammation, positive remodeling, a large necrotic core, angiogenesis, microcalcification, and a thin, fibrous cap. Each represents a potential imaging target, and although not all of these plaques will progress to rupture and even less will cause events, emerging evidence suggests that identification of such plaques may identify patients at increased cardiovascular risk. Again, CMR techniques aimed at resolving these characteristics are best suited to the carotid arteries.
Positive Remodeling
Similar black-blood imaging techniques as described earlier can be used to identify positive remodeling. Indeed, identification of positive remodeling in the aorta and carotids has been shown to identify patients with an increased risk of future adverse cardiovascular outcomes. Moreover, positive remodeling is also detectable with CMR in individual coronary arteries, although the relationship between this CMR finding and prognosis is yet to be established.
Multicontrast Magnetic Resonance
Multicontrast CMR of the plaque is based on successive high-resolution black-blood fast-spin echo CMR sequences that null signal in the flowing blood and perivascular fat with preparatory pulses. Several sequences with different weightings are generally acquired (e.g., T1 weighted, T2 weighted, and proton density weighted [PDW]). Analysis of the various signal intensities in each of these sequences allows reasonable differentiation of plaque components. In particular, the lipid core, fibrous tissue, plaque hemorrhage, the fibrous cap, and the degree of calcification can all be identified and quantified according to their different relaxation properties on CMR. In Fig. 27.1 an axial section through the thorax reveals a complex aortic plaque. Fig. 27.2 demonstrates a carotid plaque imaged at 3 T, with corresponding T1, T2, and PDW images displayed. A final example of multicontrast CMR showing different plaque components and automatic segmentation of these plaques using a k -means cluster algorithm is shown in Fig. 27.3 .
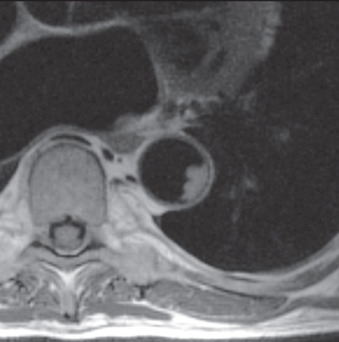
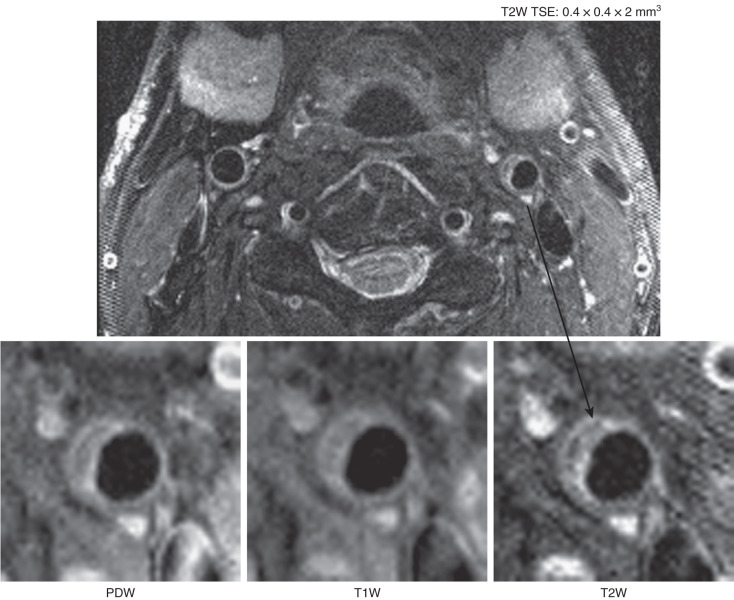
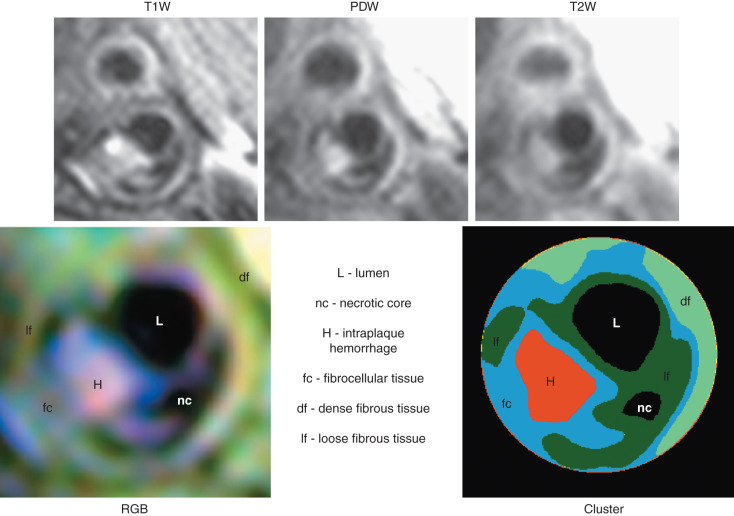
The lipid rich necrotic core can be identified in the carotid arteries and aorta using multicontrast weightings plus postcontrast T1-weighted imaging, with advances in CMR acquisition protocols allowing acquisition times to be reduced many-fold. The necrotic core usually appears isointense to hyperintense on time-of-flight angiographic and precontrast T1-weighted images and has minimal contrast enhancement compared with the surrounding tissue on postcontrast T1-weighted images. The combination of these images can therefore be used to identify and quantify the lipid necrotic core burden. Yuan et al. demonstrated that multicontrast CMR of human carotid arteries had sensitivity and specificity values of 85% and 92%, respectively, for the identification of a lipid core.
Alterations in the lipid necrotic core burden with therapy have also been demonstrated and may be more sensitive to treatment effects than simple plaque burden measurements. The ORION trial assessed the impact of rosuvastatin on carotid plaque volume and composition in 43 patients treated for 2 years. Although no change in the plaque volume was observed, a reduction in the percentage of lipid necrotic core was demonstrated. A subsequent study by Zhao and colleagues confirmed the effect of lipid-lowering therapy on the lipid content of atherosclerotic plaque after just 1 year of treatment. Importantly, the observed reduction continued in to the second year of therapy and preceded any effects on vessel wall area.
Imaging Acute Thrombus and Plaque Hemorrhage
Histopathological studies suggest that intraplaque hemorrhage may play a role in triggering both plaque rupture and growth. A first study proved that multicontrast CMR could accurately image intraplaque hemorrhage in carotid atheroma using T2*-weighted sequences. However CMR can also distinguish between recent and remote hemorrhage on the basis of its methemoglobin content using noncontrast T1-weighted gradient recalled echo sequences, incorporating fat and blood suppression. Methemoglobin is an intermediate breakdown product of hemoglobin formed 12 to 72 hours following hemorrhage. It therefore represents a key component of acute thrombus and is associated with a short T1 and high signal on T1-weighted imaging. This property allows CMR to detect fresh thrombus using T1-weighted images in a range of conditions including deep venous thrombosis and pulmonary embolism. This approach has been used in atherosclerosis to visualize regions of both intraplaque hemorrhage and endothelial thrombus related to plaque rupture/erosion. Indeed, the culprit carotid plaques of patients who have suffered a recent stroke consistently demonstrate this high T1-weighted signal.
Early Imaging of Aortic Stenosis and Calcification
Histologic validation of this approach has also been provided using the carotid model in 63 patients undergoing endarterectomy. This demonstrated that high-intensity plaques had negative and positive predictive values of 70% and 93%, respectively, for the detection of complex carotid lesions with evidence of surface rupture and intraluminal or intraplaque hemorrhage. Moreover, imaging of hemorrhage and thrombus in the coronary arteries, where the small size of the vessel wall makes multicontrast imaging challenging, are the targets of intense research (see Chapter 3 ).
Importantly, unenhanced T1-weighted CMR imaging also appears to provide important prognostic information. In one study, the presence of high-intensity plaques in the carotid arteries predicted cardiac events, outperforming carotid intimal medial thickness and traditional cardiovascular risk factors. This finding was corroborated in a subsequent meta-analysis of 8 studies incorporating data from 689 patients, which demonstrated a 6-fold increase in cerebrovascular events in patients with high-intensity carotid plaques. The approach is also applicable to the coronary arteries, with high-intensity plaque again appearing to identify patients at increased risk of future coronary events. Noncontrast T1-weighted imaging therefore holds major promise as a prognostic marker, and in studying the natural history of plaque hemorrhage and the prevalence and importance of subclinical atherosclerotic plaque rupture.
Angiogenesis
Pathological studies first revealed the central role of plaque neovascularization in contributing to atherosclerotic plaque vulnerability. The presence of neovessels is strongly associated with plaque inflammation, macrophage content, and the likelihood of rupture, presumably because they provide an alternative route for monocyte entry into the plaque and because of the propensity of these vessels to leak and rupture.
Gadolinium (Gd) chelates represent the most commonly used CMR contrast agents for imaging new plaque vessels. These paramagnetic agents increase the blood signal on CMR images after intravenous injection and are therefore good candidates for measuring plaque neovasculature. Such techniques have been used in oncology imaging to quantify new vessels associated with tumors. Kerwin and colleagues demonstrated a correlation between the increased signal intensity in carotid atheroma as a result of Gd-enhancement and the extent of plaque angiogenesis on histology. However, this imaging modality is not specific for neovascularization and is limited because Gd chelates rapidly distribute into the extracellular space. Dynamic contrast-enhanced (CE) imaging of Gd-enhancement enables tracer kinetic modeling to characterize the leakiness of the neovessels by measuring a parameter called K trans as a potential marker for plaque vulnerability. In one study, neovessel abundance was correlated histologically with Gd uptake parameters evaluated by dynamic CE CMR in a rabbit model. Relatively high reproducibility of dynamic CE imaging has been shown to allow small sample sizes. Another dynamic CE-CMR study demonstrated a reduction in plaque neovasculature with longer statin therapy. In the coronary arteries, some efforts have been made to image angiogenesis by evaluating late Gd enhancement (LGE) in the coronary artery wall. Further improvements in the quantification of plaque angiogenesis may be possible with novel intravascular albumin-binding Gd compounds that have longer half-lives in the circulation.
Fibrous Cap
Imaging late after Gd administration can also provide information about different plaque characteristics. In particular it can help differentiate between the atherosclerotic fibrous cap, which enhances with Gd, and the necrotic core that lacks its own vasculature and so appears hypointense. As discussed earlier, this approach can be used to estimate the size of the lipid-rich necrotic core. However, in addition it can be used to assess the fibrous cap in greater detail with respect to both its thickness and integrity. Indeed, LGE has been used to successfully image regions of both carotid plaque rupture and ulceration that may or may not be clinically apparent.
Three-Dimensional Plaque Imaging
As the utilization of plaque imaging has advanced, so has development of three-dimensional (3D) imaging to assess complex plaque without partial volume and re-slicing effects. Under development are 3D-imaging techniques to assess plaque burden and positive remodeling, replicate multicontrast image weighting, as well as techniques focused on single plaque components such as plaque hemorrhage.
Molecular Magnetic Resonance Imaging of Atherosclerosis
Traditional plaque imaging modalities such as computed tomography (CT) and multicontrast CMR exploit anatomical variations between tissues to provide images. Molecular (or target-specific) imaging unites vascular biology with imaging modalities such as CMR and positron emission tomography (PET), allowing us to study the activity of specific pathological processes noninvasively, and with high-spatial resolution.
As we have seen, multicontrast CMR can characterize the various plaque components of intermediate to advanced atherosclerosis by exploiting pathological changes in tissue properties that lead to modified endogenous CMR contrast. However, the use of exogenous imaging tracers targeting specific pathological processes (usually because of labeling with either Gd or iron oxide) has the potential to greatly improve the sensitivity of the technique by amplifying the CMR contrast.
Later we will discuss a variety of molecular imaging tracers that may provide improved CMR imaging of atherosclerotic plaque. We will also discuss the synergistic role of PET and the potential utility of novel hybrid PET/CMR imaging systems.
Plaque Inflammation
Resident plaque macrophages have been successfully imaged using ultrasmall superparamagnetic particles of iron oxide (USPIO). These are removed from the circulation by the reticuloendothelial system and accumulate in macrophages present in atherosclerotic plaques. Macrophages play a pivotal role in the destabilization of atherosclerotic plaques by secreting large quantities of fibrous cap-degrading metalloproteinases (MMPs), along with proinflammatory cytokines and tissue factor. Iron oxide contrast agents have superparamagnetic properties (i.e., they decrease T2* relaxation time by generating heterogeneities in the local magnetic field) and can be detected on CMR as signal voids on T2*-weighted sequences. USPIO plaque imaging with CMR has been validated against histopathology at timepoints out to 8 weeks in an animal model. Iron staining closely matched that of macrophage distribution within the plaque, but interestingly only a subset of smaller sized macrophages actively accumulated USPIO.
In clinical studies, Kooi and colleagues studied 11 symptomatic patients scheduled for carotid endarterectomy with USPIO-enhanced CMR. They reported a 24% decrease in signal intensity on corresponding T2*-weighted sequences, and histologically verified uptake of USPIO in 75% of ruptured or rupture-prone lesions. Trivedi et al. expanded on this work and demonstrated that the optimum time for imaging symptomatic carotid plaque was 24 and 36 hours after USPIO injection. USPIO imaging is also being explored in abdominal aortic aneurysm disease, on the basis that inflamed aneurysms are likely to expand more quickly and are more likely to rupture.
USPIO imaging has also been used clinically to assess the antiinflammatory effects of novel therapies. The ATHEROMA (Atorvastatin Therapy: Effects on Reduction of Macrophage Activity) study randomized 47 patients with carotid stenosis >40% and increased USPIO uptake on baseline scanning to either 10 mg or 80 mg atorvastatin therapy for 12 weeks. A significant decrease in USPIO carotid plaque uptake was observed in the high-dose cohort but not the low-dose group.
Macrophages within atherosclerotic plaques have also been targeted for imaging with Gd-loaded immunomicelles. These agents, with diameters between 20 and 120 nm, are composed of phospholipids, a surfactant, and an aliphatic chain with Gd-diethylenetriaminepentaacetic acid (Gd-DTPA) attached at the polar head group. The polar head group of the aliphatic chain can be attached to antibodies directly or via a biotin-avidin bridge. Using this model, we have made micelles that have over 10,000 Gd ions on each micelle surface and the ability to specifically target the macrophage scavenger receptor. Initial work demonstrated that these immunomicelles resulted in enhanced signal in murine atherosclerotic plaque.
Endothelial Adhesion Molecules
Expression of endothelial adhesion molecules such as VCAM and selectins occurs early in the development of atherosclerosis, driving progressive macrophage recruitment in to the plaques. These are potential imaging targets in cardiovascular disease and other conditions. The ability to image VCAM was elegantly demonstrated by Kelly et al. They used a superparamagnetic fluorescent nanoparticle coupled to a payload peptide that was internalized by endothelial cells expressing VCAM. This was tested in ApoE-/- mice fed a high cholesterol diet and was highly specific for VCAM-expressing cells, compared with immunohistochemistry.
CMR imaging of the selectin family of molecules has also been attempted, at least in vitro. Kang et al. showed that a monoclonal antibody fragment tagged with iron oxide nanoparticles was specific for human endothelial cells expressing E-selectin in culture, with an increased binding of 200 times compared with controls. Intracellular adhesion molecule 1 (ICAM-1) receptors expressed on the cerebral arterial vascular endothelium have been imaged with CMR using antibody-conjugated paramagnetic liposomes. CMR provided sufficient signal enhancement to determine the areas of increased expression, and binding was verified by fluorescent histopathology.
Angiogenesis
A novel agent targeting the integrin α v β 3 (specifically expressed on the endothelial surface of neovasculature) has been developed to identify regions in the vessel wall undergoing angiogenesis. Winter et al. demonstrated in a rabbit model of atherosclerosis that regions of neovascularization in plaques had a 47% increase in signal intensity on CMR after the injection of α v β 3 -targeted nanoparticles. Another epitope that has been successfully exploited for imaging plaque angiogenesis is fibronectin. The binding of an antifibronectin antibody was confirmed in ApoE-/- mice, both autoradiographically and by the use of a fluorescent probe, demonstrating specificity for the vasovasorum of the atheroscleorisc plaque.
Thrombus
Intraluminal thrombosis represents the final step in the evolution of vulnerable atherosclerotic plaque and is therefore a candidate target for novel-specific CMR contrast agents. Histologic studies have demonstrated that superficial thrombus superimposed on a ruptured atherosclerotic plaque characterizes those plaques at high risk of ischemic events. Moreover, a clinical study analyzed the histology of intracoronary thrombus aspirated from 211 patients with acute myocardial infarction, demonstrating that >50% of the culprit thrombi were at least days or weeks old. Presumably, microplaque rupture events with thrombus formation often predate the catastrophic rupture event that causes the clinical syndrome. The ability to detect these early warnings might therefore allow intense therapy or device placement to avert symptoms.
Aside from the T1-weighted CMR imaging approach described earlier, several molecular techniques have been taken to image thrombus with CMR. Yu et al. used a Gd-loaded nanoparticle coupled to a fibrin antibody and tested this against in vitro thrombus. They demonstrated significant signal enhancement and confirmed tight binding of the antibody to the thrombus with scanning electron microscopy. A similar method, but using a different nanoparticle construct, was employed successfully by Winter.
A fibrin-specific CMR contrast agent has also been designed. With this agent, thrombus resulting from plaque rupture has been identified using CMR in a rabbit model. In the 25 arterial thrombi induced by carotid crush injury, Botnar et al. demonstrated a sensitivity and specificity of 100% for in vivo thrombus detection. Sirol et al. used the same fibrin-specific CMR contrast agent (EP-1242) in 12 guinea pigs to demonstrate that the signal intensity of the thrombus was increased by over 4-fold compared with noncontrast images. The detection of thrombi improved from 42% pickup precontrast injection compared with 100% detection after injection. Finally, another experimental fibrin-targeted peptide (EP-2104R) for thrombus detection allowed discrimination between occlusive and nonocclusive thrombi, and tracking of intravascular thrombus as it aged and became more organized by fibrous tissue infiltration ( Fig. 27.4 ).
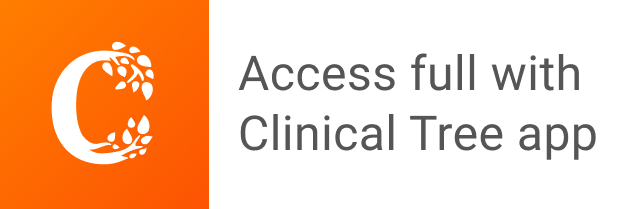