Radiopharmaceutical
Application
Indication for imaging
Radiolabeled particles
99mTc-MAA, 10–50 μm
Capillary blockade
Lung perfusion
99mTc-DTPA, aerosol, 1–4 μm
Sedimentation in bronchioles
Lung ventilation
99mTc-Sulfur colloid, 0.1–1.0 μm
Reticuloendothelial function
Liver, spleen, and bone marrow
99mTc-SC, filtered 0.1–0.3 μm
Lymphatic drainage
Breast cancer and melanoma
99mTc-HSA (nanocolloid), 0.02 μm
Lymphatic drainage
Breast cancer and melanoma
99mTc-Antimony sulfide colloid, 0.1 μm
Lymphatic drainage
Breast cancer and melanoma
Radiolabeled gases
133Xe, 127Xe, 81mKr
Alveolar transit–capillary diffusion
Lung ventilation
99mTc-Technegas, 0.004–0.25 μ
Alveolar transit–capillary diffusion
Lung ventilation
Radiolabeled chelates
99mTc-MDP, HDP
Bone formation
Metastatic bone disease, neuroblastoma, osteosarcoma
99mTc-DTPA
Blood–brain barrier disruption
Brain tumors
Renal function glomerular filtration
Renal blood flow and renogram
99mTc-MAG3
Renal function, tubular secretion
Renogram
99mTcIII-DMSA
Binding to renal parenchyma
Renal scan
99mTcV-DMSA
Tumor cell uptake
Medullary carcinoma of thyroid
99mTc-Disofenin and mebrofenin
Hepatobiliary function
Hepatobiliary imaging
99mTc-Ceretec and Neurolite
Blood flow
Brain imaging
99mTc-sestamibi and tetrofosmin
Blood flow
Myocardial perfusion
99mTc-sestamibi, and tetrofosmin
Tumor viability and multidrug resistance, MDR (Pgp expression)
Breast cancer, parathyroid adenoma, brain tumor
111In-DTPA
CSF flow
Cisternogram
111In-oxine
Radiolabeling white cells
Labeled leukocyte thrombus imaging
67Ga-citrate
Tumor viability, capillary leakage
Tumor and infection imaging
Radiotracers as ions
99mTc-pertechnetate (TcO4 –)
Thyroid function (trapping)
Thyroid imaging
123I,131I-sodium iodide (I–)
Thyroid function (trapping)
Thyroid uptake, imaging therapy
82Rb-chloride, Rb+
Blood flow
Myocardial perfusion
201Tl-thallous chloride, Tl(OH)2 +
Blood flow
Myocardial perfusion
Tumor viability
Tumor imaging (brain, parathyroid, thyroid)
Radiolabeled cells
111In-leukocytes
Cell migration and phagocytosis
Infection imaging
111In-platelets
Cell incorporation in thrombus
Thrombus imaging
51Cr-RBCs
Dilution in blood compartment
RBC mass and blood volume
99mTc-RBCs
Cardiac function
Cardiac ejection fraction, wall motion
Blood pool
Hemangioma, GI bleeding
99mTc-RBC (heat denatured)
Spleen
Accessory splenic tissue
Receptor binding radiotracers
111In-pentetreotide, Octreoscan
Somatostatin receptors
Neuroendocrine tumors
99mTc-P829, NeoTect
Somatostatin receptors
Lung cancer, NE tumors
99mTc-P280, Acutect
GP IIb/IIIa receptors
Thrombus imaging, DVT
99mTc-TRODAT-1
Dopamine transporter
Brain imaging-dopamine D2 receptors
123I-VIP
VIP receptors
Gastrointestinal tumors
131I-NP-59
LDL receptor, cholesterol metabolism
Adrenal carcinoma, adenoma, Cushing’s syndrome
123I- or 131I-MIBG
Presynaptic adrenergic receptors
Myocardial failure
Adrenergic tissue uptake
Tumor imaging (pheochromocytoma, neuroendocrine, neuroblastomas)
[11C]Raclopride
Dopamine D2 receptors
Brain imaging-dopamine D2 receptors
123I-IBZM
Dopamine D2 receptors
Brain imaging-dopamine D2 receptors, tumor imaging, malignant melanoma
[18F]fluoro-estradiol (FES)
Estrogen receptors
Breast tumor imaging
Radiolabeled monoclonal antibodies
111In-OncoScint, B72.3 IgG
TAG-72 antigen
Colorectal and ovarian cancer
111In-ProstaScint, 7E11-C5.3 IgG
PSMA (intracellular epitope)
Prostate cancer
99mTc-CEA-Scan, IMMU-4 Fab’
CEA
Colorectal cancer
99mTc-Verluma, NR-LU-10 Fab’
Cell surface GP as antigen
Small-cell lung cancer
99mTc-fanolesomab (CD15)
Granulocyte antigen CD15
Appendicitis
111In-antimyosin
Antimyosin
Acute myocardial infarction, heart transplant rejection
Radiolabeled metabolic substrates
18F-fluorodeoxyglucose, FDG
Tumor viability and metabolism
Tumor imaging
Glucose metabolism
Brain and cardiac imaging
18F-fluorothymidine
Cell proliferation
Tumor imaging and monitoring treatment
11C-choline
Cell proliferation
Brain tumors
[11C] or 123I-methyltyrosine
Protein synthesis, protein upregulation
Brain tumors
11C-methionine
Amino acid transport
Brain and pancreatic tumors
[11C]-thymidine
DNA synthesis, cell proliferation
Brain tumors
[18F] and 123I-fatty acids
Myocardial metabolism
Cardiac imaging
[57Co]-vitamin B12
Vitamin B12 absorption
Pernicious anemia
18F-fluoromisonidazole
Hypoxia and oxidative metabolism
Tumors selected for radiotherapy
18F-fluoroethyltyrosine(FET)
Amino acid transporter
Brain tumors
Radiopharmaceutical agents exhibit a huge range of physical and chemical properties and may be classified into different categories. The most important factors that influence the transport, uptake, and retention of radiopharmaceuticals in different organs and tissues include the chemical and biochemical nature of the carrier molecule transporting the radionuclide of choice to the targeted area.
The use of radiopharmaceuticals to deliver therapeutic doses of ionizing radiation has been extensively investigated. Targeted radionuclide therapy by systemic administration of a radiopharmaceutical provides a potential to treat widely disseminated cancer tissue. A number of radiopharmaceuticals (Table 3.2) are now available for the treatment of different malignancies or palliation of pain due to bony metastases. Tumor-specific radiopharmaceuticals that are clinically useful for noninvasive imaging of tumors are being modified for radionuclide therapy of tumors.
Table 3.2
Radiopharmaceuticals for therapy
Radiopharmaceutical | Application | Specific tumors |
---|---|---|
131I-sodium iodide | Thyroid function | Differentiated thyroid carcinoma |
131I-MIBG | Adrenergic tissue | Colorectal cancer metastatic to liver and bladder cancer |
131I-anti-B1 antibody | Anti-CD22 antigen | Lymphoma |
90Y-MXDTPA-anti-B1 antibody | Anti-CD22 antigen | Lymphoma |
32P-chromic phosphate (colloid) | Cell proliferation and protein synthesis | Peritoneal metastases, recurrent malignant ascites |
32P-orthophosphate | Cell proliferation and protein synthesis | Polycythemia vera |
89Sr chloride | Exchanges with Ca in bone | Palliation of pain due to bony metastases |
153Sm-EDTMP | Binds to hydroxyapatite | Palliation of pain due to bony metastases |
117mSn-DTPA | Binds to hydroxyapatite | Palliation of pain due to bony metastases |
186Re-HEDP | Binds to hydroxyapatite | Palliation of pain due to bony metastases |
90Y-DOTA-Tyr3-octreotide | Somatostatin receptors | Neuroendocrine tumors |
90Y-DOTA-lanreotide | Somatostatin receptors | Neuroendocrine tumors |
90Yb-ibritumomab | Lymphocyte antigen CD20 | Lymphoma |
3.2 Mechanism(s) of Radiopharmaceutical Localization
The uptake and retention of radiopharmaceuticals by different tissues and organs involve many different mechanisms, as summarized in Table 3.3. The pharmacokinetics, biodistribution, and metabolism of the radiopharmaceutical are very important to understanding the mechanisms of radiopharmaceutical localization in the organ or tissue of interest. Injury to a cell or tissue significantly alters the morphology and molecular biology compared with that of normal tissue or organs.
Table 3.3
Mechanisms of radiopharmaceutical localization
Mechanism | Radiopharmaceutical |
---|---|
1. Isotope dilution | 125I-HSA, 51Cr-RBC, and 99mTc-RBC |
2. Capillary blockade | 99mTc-MAA |
3. Physicochemical adsorption | 99mTc-MDP, HDP |
4. Cellular migration | 111In- and 99mTc-leukocytes, 111In-platelets |
5. Cell sequestration | Heat denatured 99mTc-RBC |
6. Simple diffusion | 133Xe, 81mKr, 99mTc-pertechnegas |
Diffusion and mitochondrial binding | 99mTc-sestamibi and tetrofosmin |
Diffusion and intracellular binding | 99mTc-Ceretec and Neurolite |
Diffusion and increased capillary permeability | 67Ga-citrate |
7. Facilitated diffusion and transport, protein upregulation | [18F]-FDG, radiolabeled amino acids |
8. Active transport | Radioiodide, 99mTcO4 –, 201Tl thallous cation |
[18F]-FDG, radiolabeled amino acids | |
Na+/K+ ATPase pump | 201Tl thallous cation |
9. Phagocytosis | 99mTc-colloids in RES and lymph nodes |
10. Increased vascular permeability and capillary leakage | 67Ga-citrate, radiolabeled proteins |
11. Cell proliferation | [11C]-thymidine, [124I]-iododeoxyuridine (IudR), 18F-fluorothymidine (FLT) |
12. Metabolic trapping | [18F]-FDG, 99mTc-pertechnetate |
13. Metabolic substrates | 123I and 131I as sodium iodide, 123I-fatty acids |
14. Tissue hypoxia and acidic pH | [18F]fluoromisonidazole, 67Ga-citrate |
15. Specific receptor binding | |
Somatostatin receptors | Octreoscan, NeoTect |
VIP receptors | 123I-VIP |
Transferrin receptors | 67Ga-citrate |
Estrogen receptors | 16α-[18F]fluoro-17β-estradiol (FES) |
Dopamine D2 receptors | [123I]-IBZM, 99mTc-TRODAT |
LDL receptors | 131I-6β-iodomethyl-19-norcholesterol (NP-59) |
Presynaptic adrenergic reuptake | [131I or 123I]-MIBG |
16. Specific binding to tumor antigens | |
17. PSMA | ProstaScint |
CEA | CEA-Scan |
TAG-72 | OncoScint |
Cell surface 40-kd glycoprotein | Verluma |
CD22 | Bexaar |
CD15 | 99mTc-fanolesomab |
Antimyosin | 111In-antimyosin |
The mechanisms of radiopharmaceutical localization may be substrate nonspecific (not participating in any specific biochemical reaction) or substrate specific (participating in a specific biochemical reaction), depending upon the chemistry of the molecule. Many radiopharmaceuticals were designed to take advantage of the pathophysiology in order to increase the specificity of the nuclear medicine imaging techniques. Since some radiopharmaceuticals are not specific for a particular disease, the cellular uptake might include a combination of different mechanisms, as in the case of 67Ga-citrate. However, the unique chemistry of each radiopharmaceutical may determine the manner in which it is transported and retained within a specific tissue or organ. It is very important to recognize that since the radiopharmaceutical may undergo significant metabolism and degradation in vivo, the observed biodistribution and tissue localization may represent the behavior of only radiolabeled metabolic product and not necessarily that of the intact parent radiopharmaceutical as exemplified by 99mTc l,l-ECD. For the radiopharmaceuticals that do not undergo biotransformation the biodistribution and tissue localization represent the behavior of the parent radiopharmaceuticals such as 99mTcMDP, 99mTc colloids, and gaseous radiopharmaceuticals.
In addition, the patient’s medication, physical status, and other factors may significantly alter the biodistribution and tissue localization and retention characteristics of a radiopharmaceutical. The different mechanisms of localization are discussed below, using specific examples of the more common radiopharmaceuticals.
3.2.1 Isotope Dilution
The dilution principle is based on the concept of “diluting” a radiotracer (or tracer) of known activity (or mass) in an unknown volume. By measuring the degree to which the radiotracer was diluted by the unknown volume, one can determine the total volume (or mass) of the unknown volume. The dilution principle is currently used for a quantitative determination of RBC volume (mass), plasma volume, and total blood volume.
It is very important that the radiotracer remain only in the blood volume to be measured. Nondiffusible intravascular agents such as 51Cr-RBCs are used to measure RBC mass, while 125I-HSA is used to measure plasma volume. There is no specific mechanism involved other than simple dilution of the radiotracer.
3.2.2 Capillary Blockade
The technique most commonly used to determine the perfusion to an organ depends on trapping the radiolabeled particles (microembolization) in the capillary bed of an organ such as lung, heart, or brain. Following intravenous injection, 99mTc-MAA particles are physically trapped in the arteriocapillary beds of the lung and block the blood flow to the distal regions. Therefore, the mechanism of localization of particles in lungs is purely a mechanical process, called capillary blockade.
3.2.3 Physicochemical Adsorption and Ion Exchange
Bone scanning with 99mTc-labeled phosphonates (MDP, HDP) is extensively used in nuclear medicine to evaluate benign and malignant bone diseases. The 99mTc-phosphonates accumulate in the hydroxyapatite (HA) crystal (containing Ca2+ and phosphate ions) matrix or in the amorphous (noncrystalline) calcium phosphate (ACP).
The principal uptake mechanism of the radiotracer appears to be simply “physicochemical adsorption.” However, the exact mechanisms involved in the extraction of the radiotracer from the blood through the endothelial cells, extracellular fluid, and finally to HA are not known. In contrast to the P–O–P bond in phosphates, the P–C–P bond in phosphonates is not a substrate for alkaline phosphatase and is very stable in vivo.
Primary bone tumors such as osteogenic sarcomas avidly accumulate bone agents because of the production of bone matrix in extraosseous tissue. Metastatic deposits that produce a vigorous osteoblastic response will appear as hot spots in a bone scan, while the lesions that generate osteolytic reactions may not accumulate the bone agent [3]. The bone scanning agents may also be taken up occasionally in soft tissues. The primary underlying factor responsible for the uptake of these tracers is excess calcium in soft tissue. Cell hypoxia and cell death would lead to increased deposition of calcium phosphates in the extracellular fluid. The uptake of 99mTc-phosphonates in the soft tissues is believed to be due to chemisorption on the surface of calcium salts such as in myocardial infarction.
The localization of bone-seeking radiotracers in increased amounts at the tumor–bone interface provides the basis for the use of radionuclides in the treatment of bone pain. Several radiopharmaceuticals (Table 3.3) are indicated for relief of pain (bone pain palliation) in patients with confirmed osteoblastic bone lesions. The exact mechanism of action of relieving the pain of bone metastases is not known, however.
For the ion exchange mechanism in the use of 18F-NaF, the fluoride ion exchanges with the hydroxyl ion in the hydroxyapatite crystal of bone to form fluorapatite [4]. It is therefore conceivable that in addition to imaging primary and metastatic tumors 18F can be used for studying bone metabolism.
3.2.4 Cellular Migration and Sequestration
111In-oxine- or 99mTc-HMPAO-labeled autologous mixed leukocytes (predominantly neutrophilic polymorphonuclear leukocytes, PMNs) are routinely used to image various inflammatory diseases and infectious processes. The inflammatory reaction is a well-described sequence of events (see Chap. 4) in response to an infection.
In an acute infection, the predominant cells infiltrating a site of infection are the PMNs. Following intravenous administration of radiolabeled leukocytes, the labeled cells migrate to the site of infection, similar to the circulating leukocytes because they are attracted by the immediately generated chemotactic factors, such as complement subcomponents.
In a similar manner, 111In-platelet localization at the site of active thrombus formation also involves simple cellular migration, since platelets play a major role in thrombus formation. Accessory splenic tissue can develop after splenectomy. Heat-damaged 99mTc-RBCs are more specific for the detection of accessory splenic tissue. Following intravenous administration, the spleen sequesters the heat-damaged RBCs in the same way that old and damaged circulating RBCs are normally removed. Hence, the heat-damaged 99mTc-RBCs can be used to visualize the spleen when 99mTc colloid fails to do so [5, 6].
3.2.5 Membrane Transport
Transport through the lipid bilayer or through the transport proteins may involve simple diffusion, passive transport (facilitated diffusion), or active transport mechanisms. Certain macromolecules may also be transported by vesicle formation, involving either endocytosis or exocytosis mechanisms.
3.2.5.1 Simple Diffusion
The mechanism of localization of many radiopharmaceuticals in target organs involves a simple diffusion process. The direction of movement of the radiotracers by diffusion is always from a higher to a lower concentration, and the initial rate of diffusion is directly proportional to the concentration of the radiotracer. A net movement of molecules from one side to another will continue until the concentration on each side is at chemical equilibrium. The gases used for ventilation studies, such as 133Xe, 127Xe, and 81mKr, are inert lipophilic gases. Following their administration through inhalation, these gases are distributed within the lung air spaces by diffusion, proportional to ventilation. The distribution, however, is interrupted in obstructive airways. The gases pass from the lungs into the pulmonary venous circulation and are released through the lungs by the mechanism of alveolar capillary diffusion. Similarly, distribution of 99mTc-Technegas within the lung also involves diffusion. By contrast, the irregular distribution of 99mTc-DTPA aerosol preparation within the lung is due mostly to gravity sedimentation depending on particle size.
Simple Diffusion and Intracellular Biotransformation
The blood–brain barrier (BBB) plays an important role in the mechanism of localization of many radiopharmaceuticals in the brain. The endothelial cells of the cerebral vessels form a continuous layer without gap junctions, preventing diffusion of water-soluble molecules.
In certain pathological conditions, the BBB is disrupted, allowing water-soluble molecules to diffuse from the blood into brain tissue. Traditionally, brain scan was performed with such radiopharmaceuticals as 99mTc-pertechnetate and 99mTc-DTPA that diffuse freely in the extracellular fluid and can accumulate in lesions with defects in BBB. The intact BBB does allow the transport of small molecules across the plasma membrane of the neuron by facilitated diffusion. Some small, neutral molecules, however, can cross the BBB depending on their relative lipid solubility. Brain perfusion-imaging agents such as 99mTc-HMPAO and 99mTc-ECD are lipophilic radiotracers that cross the BBB via passive diffusion. The extraction of these tracers by the brain tissue is proportional to regional cerebral blood flow (rCBF). The retention of these tracers within the neuronal tissue following diffusion and extraction is assumed to be due to intracellular biotransformation to polar metabolites or charged complexes that cannot be washed out of the cell by back-diffusion as exemplified by the cellular retention of 99mTc-ECD (Fig. 3.1) and 99mTc HMPAO.
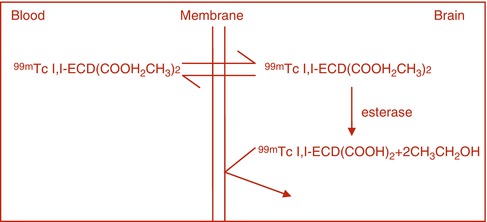
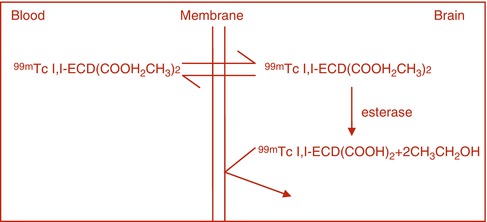
Fig. 3.1
With the labeling of red blood cells (RBCs) with 99mTc whether in vitro or in vivo, the 99mTcO4 freely diffuses in and out of the RBCs, but in the presence of stannous ion it is reduced intracellularly, where it reacts with hemoglobin (HB) to form 99mTc-Hb. The 99mTc-Hb does not diffuse out of the RBCs as shown in Fig. 3.2
For 99mTC-ECD the radiotracer freely diffuses into the brain tissue, where it is hydrolyzed by the action of esterase to a carboxylic acid which is trapped in the brain tissue.
For 99mTcHMPAO, it is presumed that the tracer is protonated to form a positively charged molecule (the so-called secondary 99mTc HMPAO) that is trapped in the brain tissue.
Similarly, in vivo labeling of ROCs with 99mTc pertechnetate occurs by independent simple diffusion of 99mTc pertechnetate and stannous ion into the red blood cell where the stannous ion reduces the pertechnetate and the reduced 99mTc binds to Hgb to form 99mTc Hgb that does not diffuse out of the red cell (Fig. 3.2).
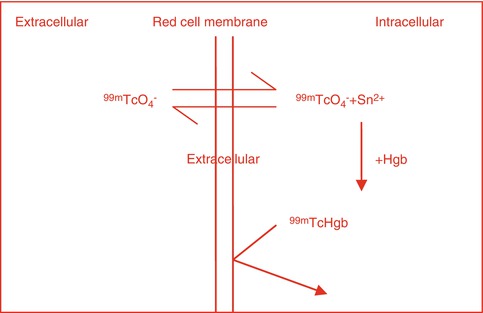
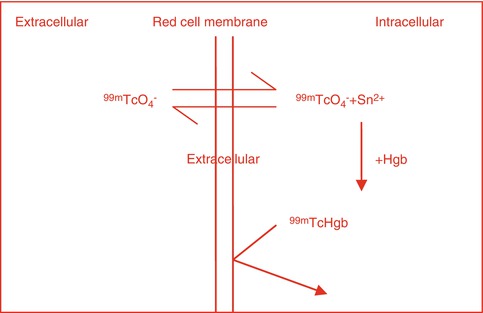
Fig. 3.2
Intracellular binding of 99mTcO4 to Hgb
Simple Diffusion and Mitochondrial Binding
A number of lipophilic, cationic 99mTc radiopharmaceuticals (sestamibi, tetrofosmin, and furifosmin) have been developed for imaging myocardial perfusion. Although 99mTc-sestamibi is cationic, similar to 201Tl+, the transport of this agent through the cell membrane involves only passive diffusion since the transport process is temperature dependent and nonsaturable [7].
The myocardial cell uptake of 99mTc-sestamibi is due to intracellular binding of 99mTc-sestamibi associated mainly with mitochondria. The cellular entry of 99mTc-sestamibi is related to the mitochondrial metabolism and the negative inner membrane potential of the mitochondria [8]. Hematochondrial retention of 99mTc-sestamibi, however, is not organ or tumor specific, but appears to be a mechanism common to most types of tissue. The intracellular levels of Ca2+ in normal cells are significantly low. However, with irreversible ischemia, extracellular calcium enters the cell and is sequestered in the mitochondria, resulting in mitochondrial destruction. The increased calcium concentration in mitochondria blocks 99mTc-sestamibi binding to mitochondria.
99mTc lipophilic cationic complexes are also used for diagnostic imaging of parathyroid adenomas, osteosarcomas, and tumors of the brain, breast, lung, and thyroid. The mechanisms of uptake of sestamibi and tetrofosmin in tumors are different from that of thallium-201 [9]. While the 99mTc agents are associated with mitochondria, 201Tl remains in the cytoplasmic compartment.
There are significant differences between sestamibi and tetrofosmin regarding intracellular localization based on in vitro studies. While 90 % of total sestamibi was associated with mitochondria, most of the tetrofosmin accumulated in the cytosolic fraction [9].
The transport of the tracer out of the tumor cell is mediated by P-glycoprotein (Pgp), a 17-kd plasma membrane lipoprotein encoded by the human multidrug resistance (MDR) gene. Piwnica-Worms et al. [10, 11] demonstrated that sestamibi is a transport substrate for Pgp and is useful for imaging Pgp expression.
Simple Diffusion and Increased Capillary and Plasma: Membrane Permeability
67Ga-citrate has been shown to localize in a variety of tumors and inflammatory lesions. There is still no general agreement on the exact mechanisms of localization in tumors [12, 13]. Following intravenous administration of carrier-free 67Ga as gallium citrate, 67Ga is bound exclusively to the two specific metal-binding sites of iron transport glycoprotein, transferrin in normal plasma, and is transported to normal tissues and tumor sites predominantly as 67Ga–transferrin complex [14].
The mechanisms involved in the uptake of 67Ga by tumor cells are very complex, since a variety of factors appear to affect transport and retention of 67Ga within the tumor tissue. Based on in vivo studies, Hayes et al. [15] concluded that the initial entry of 67Ga into tumor tissue involves simple diffusion of the unbound or loosely bound form of 67Ga, whereas its uptake by normal soft tissues is strongly promoted by its binding to transferrin. There is increased transferrin concentration within the interstitial fluid of the tumors. The increased permeability of the tumor cell membrane compared with normal cells may also account for increased diffusion of non-transferrin-bound gallium species into cells. The accumulation of 67Ga within tumor cells is very much dependent upon the intracellular binding of 67Ga to iron-binding proteins such as lactoferrin and ferritin or other higher-molecular-weight molecules which can chelate gallium with greater affinity, thereby preventing back-diffusion of free gallium species [13].
3.2.5.2 Facilitated Diffusion
Facilitated diffusion is also called carrier-mediated diffusion because a substance transported in this manner usually cannot pass through the membrane without a specific carrier protein allowing its entry. That is, the carrier facilitates the diffusion of the substance to the other side. However, the carrier protein transports a specific molecule partly but not all the way through the membrane. The molecule to be transported enters the channel and then becomes bound. Then in a fraction of a second a conformational change occurs in the carrier protein so that the channel now opens to the opposite side of the membrane. This mechanism allows the transported molecule to diffuse in either direction through the membrane as shown in Fig. 3.3.
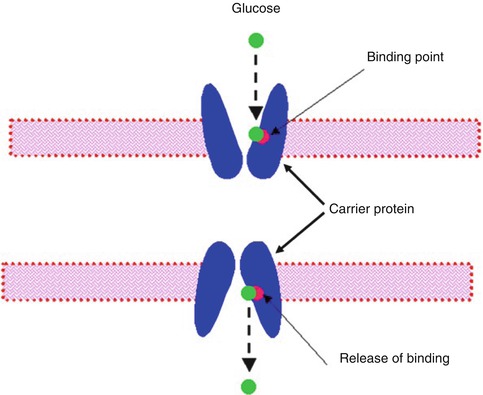
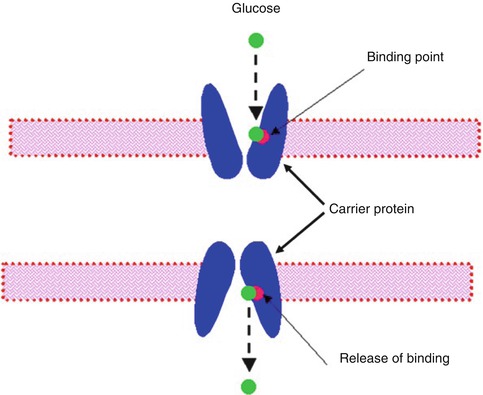
Fig. 3.3
A postulated mechanism for facilitated diffusion
18F-fluorodeoxyglucose (FDG)
The glucose analog deoxyglucose, which has one oxygen atom less than the glucose molecule (Fig. 3.4), is transported into the cell in the same way as glucose. The uptake of glucose by human cells can take place however through two mechanisms of transport. The first method involves the facilitative glucose transport (GLUT) and the second method is the active transport.
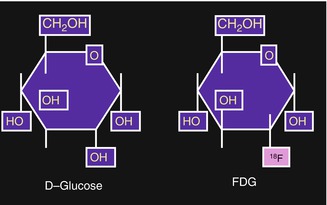
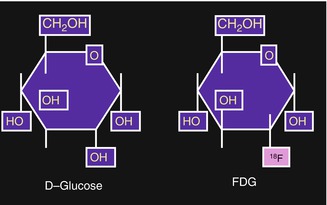
Fig. 3.4
[18F]2-deoxy-2-fluoro-d-glucose (FDG). In FDG the hydroxyl group in the 2-position of d-glucose is replaced by 18F
Six isoforms of facilitative glucose transporters have been identified so far: GLUT-1 to GLUT-5 and GLUT-7 (Table 3.4). These isoforms share the same transmembrane topology, but they differ in kinetic properties, tissue location, sugar specificities, and regulation in states of imbalanced glucose homeostasis [16].
Table 3.4
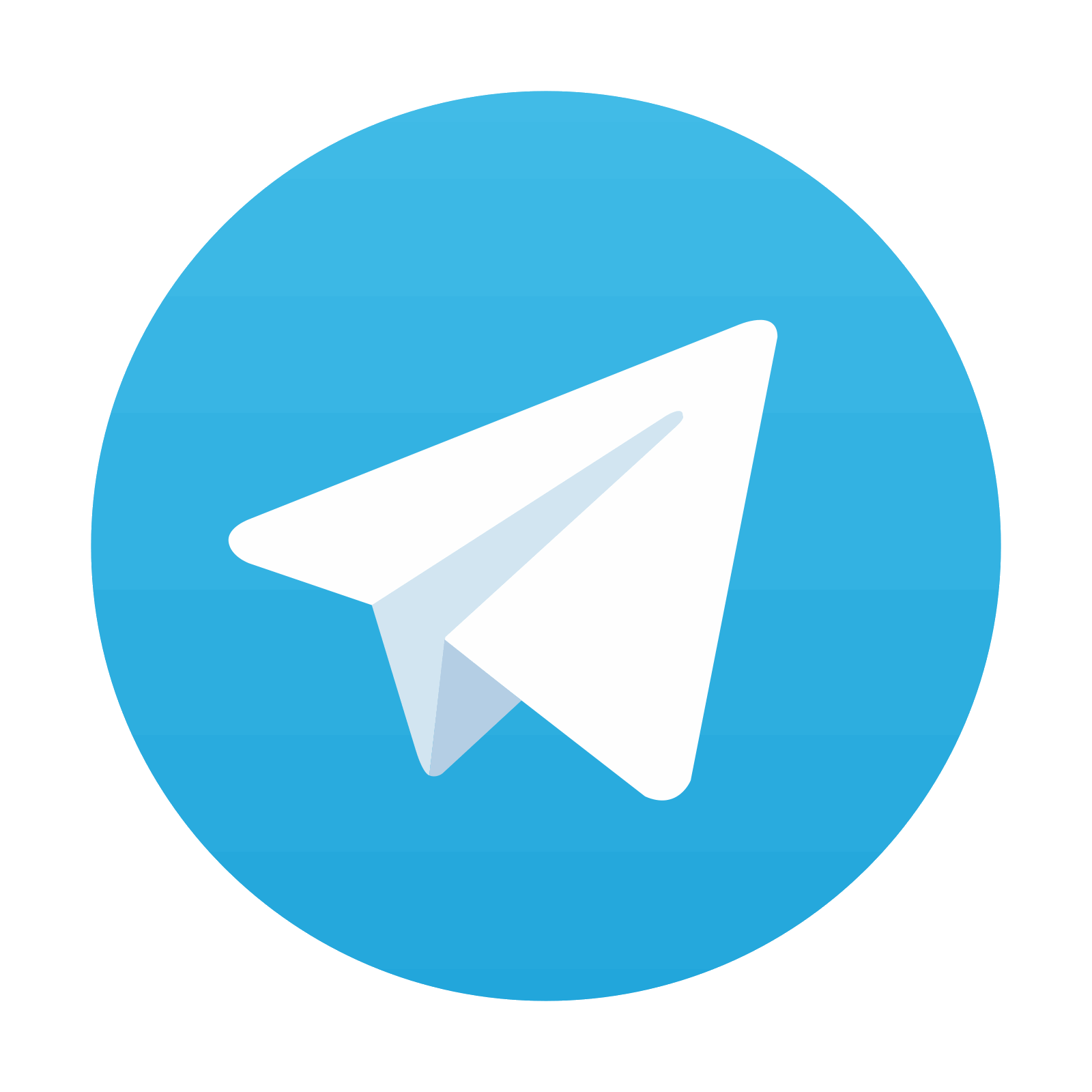
Facilitative glucose transporters and their tissue locations
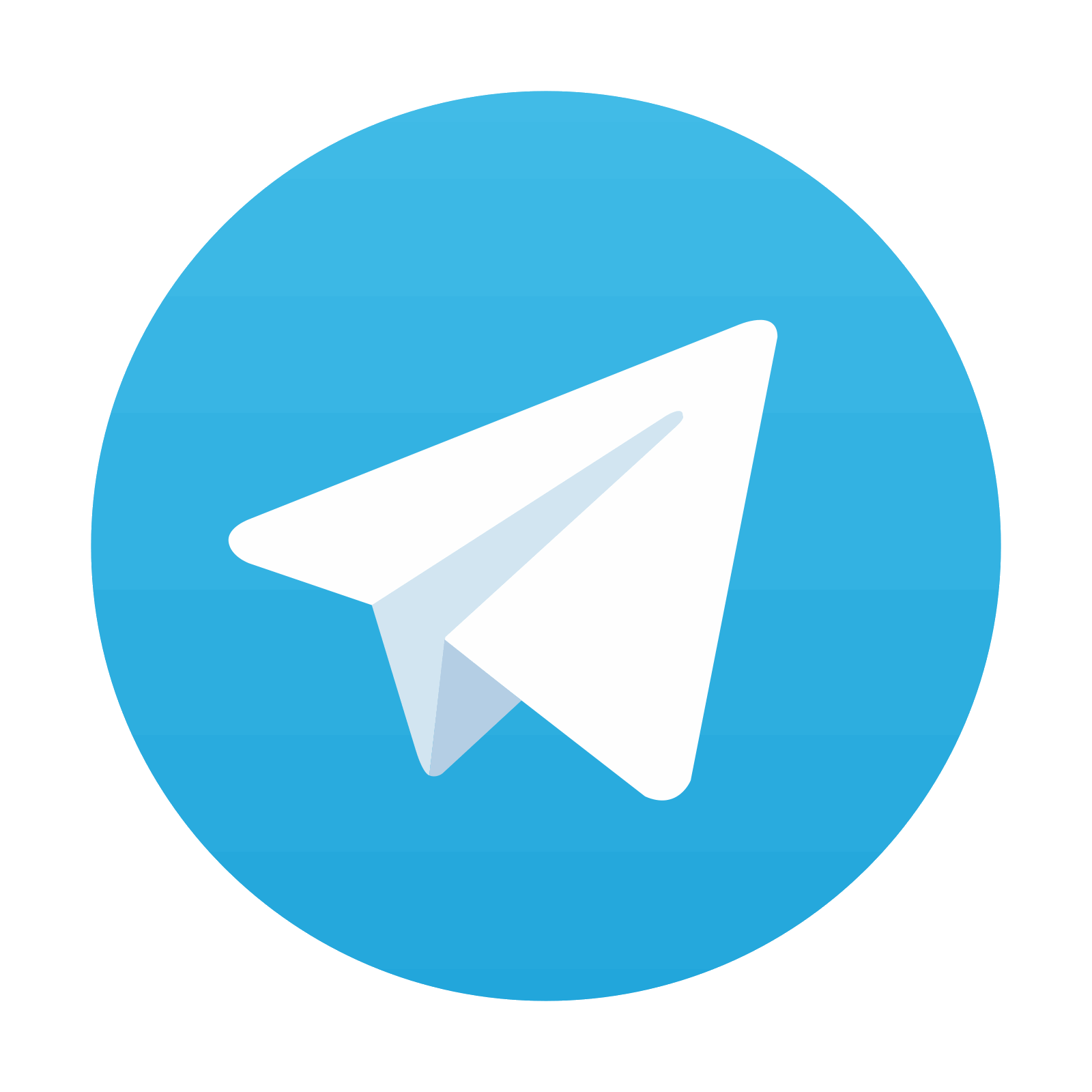
Stay updated, free articles. Join our Telegram channel
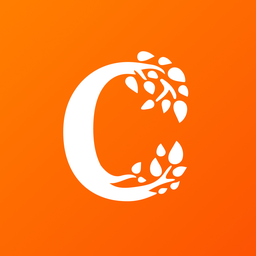
Full access? Get Clinical Tree
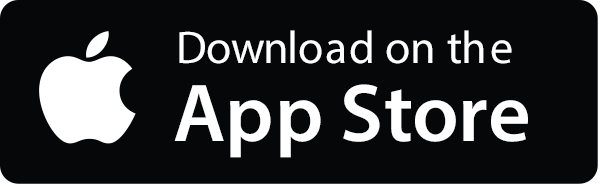
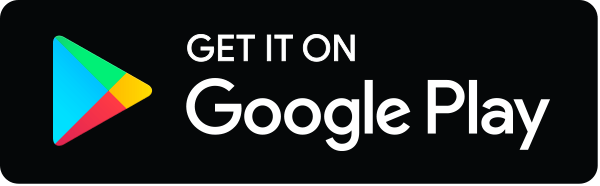
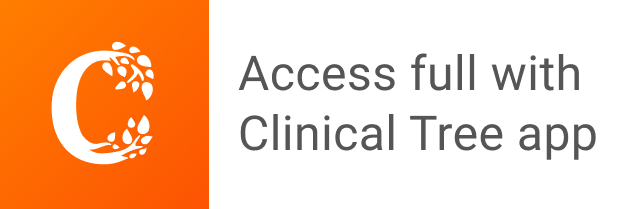