Left ventricular (LV) diastolic function has been recognized as an important factor in the pathophysiology of many common cardiovascular diseases. Dilated and hypertrophic cardiomyopathy (HCM), coronary artery disease (CAD), and systemic hypertension are all associated with abnormal LV filling dynamics. Diastolic dysfunction has also been increasingly appreciated as a major cause of heart failure (HF), especially in the elderly. Although invasive hemodynamic measures/assessment of diastole are considered the gold standard, echocardiographic methods, including tissue Doppler imaging, have gained greater use in the clinical assessment of LV diastolic function because of their noninvasive acquisition, which greatly facilitates serial assessments. In cardiovascular magnetic resonance (CMR) imaging, with the advent of parallel imaging, three-dimensional (3D) data collection, spiral and balanced steady-state free precession (bSSFP) imaging, and higher magnetic field strength, the prolonged tag persistence permits easier access to diastole. Together with rapid state-of-the-art software analysis tools, quantification of LV diastolic wall motion can now easily be performed using CMR.
Cardiac Motion
Systolic and Diastolic Heart Motion
During systole, the heart performs a complex motion analogous to “wringing” a towel, and as a result, the base and apex rotate in opposite directions. There is counterclockwise rotation at the apex and clockwise rotation at the base. In parallel, the atrioventricular valvular plane (basal left ventricle and right ventricle) descends toward the apex. The lateral free wall of the right ventricle performs a more pronounced long – axis contraction than the lateral wall of the left ventricle. During isovolumic relaxation, the myofibrils return to their resting state from the contracted state. This process is accompanied by a rapid untwisting at the apex, whereas the volume and cavity shape of the heart remain nearly unchanged. This rapid untwisting typically lasts less than 75 ms and precedes the early passive filling phase of the ventricles. During this filling phase, practically no rotational components can be seen at the apex of the healthy heart.
Understanding different diastolic strain components and their intrinsic mechanisms during different pathologic conditions allows for better understanding of diastolic dysfunction. Isovolumic relaxation is associated with changes in principal strains and untwisting, which are all related to an apparent increase in the LV volume, likely reflecting expansion of the LV myocardium. The diastolic shear strain rates are linearly related to the corresponding preceding systolic shear strain components. Nevertheless, the torsional recoil is uncoupled from end-systolic volume or associated strains. In normal subjects, the circumferential-longitudinal shear strain decreases before the axial strain. Furthermore, the early-diastolic filling efficiency can be augmented during exercise stress in an effort to maintain stroke volume despite shortened diastole. Studying early-diastolic strain patterns through myocardial strain analysis allows for comparing normal subjects with those with diastolic dysfunction. Therefore clinicians would be able to identify patients with regional diastolic dysfunction that places them at the risk of HF and sudden cardiac death following pathologic conditions such as myocardial infarction (MI), and aortic stenosis (AS).
Assessment of Cardiac Rotation/Motion: Noncardiovascular Magnetic Resonance Methods
With echocardiographic imaging, the myocardium has relatively poor internal structure because of the absence of structural landmarks, making limited quantification of parameters, such as rotation, stress, and strain. Several invasive methods have been reported during diastole to examine cardiac motion. One approach is the surgical/invasive implantation of tantalum markers into the midwall of the myocardium. In combination with x-ray angiography, the motion of these markers can then be recorded with high temporal and spatial resolution. Using such an approach, alterations in diastolic untwisting have been observed in patients who have undergone heart transplantation shortly before rejection. Although this method is very powerful, it is invasive, requires ionizing radiation, and is inappropriate for routine and repeated clinical use. Alternative angiographic “markers,” such as tracking of the bifurcations of the coronary arteries, suffer from the limited number of landmarks and their irregular geometric distribution. Furthermore, they only provide motion information about the epicardial layers of the myocardium.
Assessment of Cardiac Rotation/Motion: Cardiovascular Magnetic Resonance Methods
Similar to echocardiography, conventional cine CMR images do not provide information about the internal structure of the myocardium. However, a CMR myocardial tagging technique, spatial modulation of magnetization (SPAMM), originally proposed by Axel and further developed and refined by others, offers the opportunity to assess strain noninvasively. With these methods, the magnetization of the muscle tissue is spatially modulated, or “tagged,” by the application of a specific time series of radiofrequency (RF) pulses and magnetic field gradients. The tagging is typically applied immediately after the R-wave of the electrocardiogram (ECG). Images are then acquired during successive heart phases in which the tags may be identified as dark lines or grids. Because these tags are spatially fixed with respect to the muscle tissue at the time of the tag’s application, local myocardial motion can be derived from the translation, rotation, and distortion of the tags on the myocardium. However, because of the relaxation effects, the tags fade rapidly and cannot be reliably detected after end systole (approximately 300 ms after tag application). This is a serious drawback for the quantification of systolic and diastolic dynamics of the heart wall. Another limitation is that this approach does not compensate for through-plane motion. These limitations have been resolved through the development of the complementary SPAMM (CSPAMM) technique, on which we will focus in the remainder of the chapter.
Complementary Spatial Modulation of Magnetization: Technical Developments
Complementary Spatial Modulation of Magnetization Tagging
One limitation of SPAMM tagging is the fading of the tagging pattern through the cardiac cycle as a result of longitudinal magnetization relaxation. The loss of tagging contrast toward the end of the cardiac cycle results in unrecognizable tagging pattern, which precludes the analysis of diastolic heart phases. It was not until 1993 when Fischer et al. introduced the CSPAMM sequence to resolve this problem ( Fig. 22.1 ). To grasp the idea behind CSPAMM, it is necessary to understand the magnetization evolution with time in a tagging sequence. Immediately after applying the tagging module of the pule sequence, the whole magnetization is tagged or modulated (90-degree RF pulses are assumed here) and stored in the longitudinal position. With time, the magnetization experiences longitudinal relaxation, trying to reach the equilibrium state. This has two effects on the stored tagging pattern: (1) introducing a growing nontagged magnetization offset (we call it here the DC component, borrowing the term direct current [DC] from electrical engineering); and (2) reducing the magnitude of the tagged component (the peak-to-peak difference of the sinusoidal tagged magnetization). Thus, during the imaging stage, the excited magnetization has two components: tagged and DC, with the DC overhead impairing the visibility of the (already fading) tagged component. It should be noted that the multiple applications of RF pulses during the imaging (data acquisition) part of the sequence also contributes to reducing the tagged magnetization component (each RF pulse consumes part of the tagged magnetization stored in the longitudinal direction). The solution provided by CSPAMM consisted of two parts: eliminating the nontagged (DC) magnetization and enhancing the fading tagged magnetization. To eliminate the nontagged magnetization, two consecutive SPAMM scans are acquired with exactly the same parameters, except for the polarity of one of the tagging RF pulses. The 90-degree/90-degree tagging RF pulses in the first scan modulate the magnetization with a positive sinusoidal pattern, whereas the 90-degree/−90-degree RF pulses in the second scan result in a negative sinusoidal pattern. Note that the DC magnetization component is the same in both scans at corresponding time points. Therefore, the overhead DC magnetization can be simply eliminated by subtracting the images in the first scan from the corresponding images (at the same heart phases) in the second scan. This subtraction has also the effect of improving the image’s signal-to-noise ratio (SNR) by 40% as two acquisitions with independent noise terms are added together. To resolve the second problem of fading tagging contrast, the concept of “ramped flip angle” was introduced. Basically, during the imaging stage, the flip angles of the RF pulses determine how much magnetization is tipped into the transverse plane for data acquisition. Thus increasing the flip angles through the cardiac cycle compensates for the fading tagging contrast as a larger percentage of the available longitudinal magnetization is used at later heart phases.
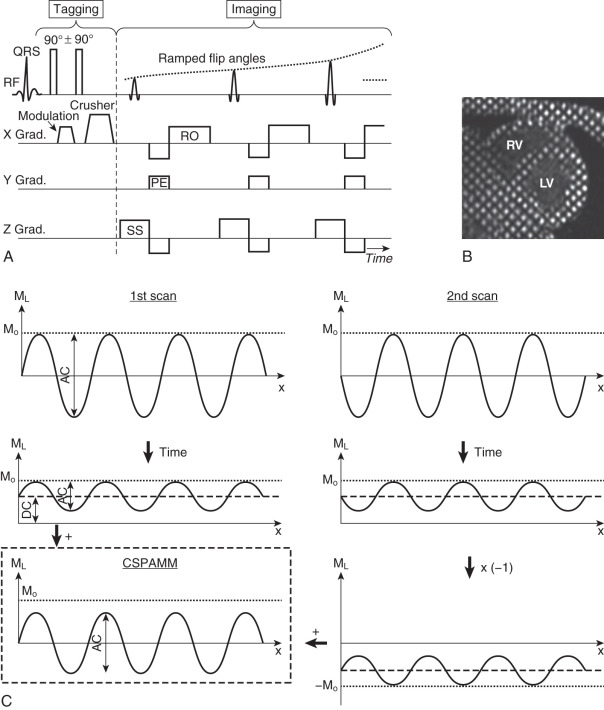
Complementary Spatial Modulation of Magnetization With Slice-Following
Because of through-plane cardiac motion, two-dimensional (2D) cine imaging of the heart may not show the same myocardial tissue throughout the cardiac cycle; rather, the imaging plane shows whatever tissue lies inside it at the time of data acquisition. This could lead to inaccurate assessment of myocardial motion; for example, an apparent myocardial thickening in a basal short-axis slice could be in fact caused by myocardial basal displacement toward the apex. Slice-following CSPAMM was developed as an improvement of the original CSPAMM technique to resolve the through-plane motion problem. The technique is based on implementing slice-selective tagging instead of the nonselective tagging used in conventional CSPAMM. A thin slice of interest is tagged by switching one (or both) of the tagging RF pulses with a slice-selective pulse, which has the effect of confining the tagging pattern inside the slice of interest (whose slice thickness = Δ z , as shown in Fig. 22.2 ). Later, during the data acquisition part of the sequence, a thicker slice (whose slice thickness of Δ s encompasses the thin tagged slice) is excited. The excited slice should be thick enough to accommodate the thin tagged slice despite its displacement in the through-plane ( z ) direction. Because nontagged magnetization is eliminated in CSPAMM, the only source of signal in the resulting image comes from the initially tagged slice, regardless of its displacement in the through-plane direction. This ensures that the same myocardial tissue is imaged during the whole cardiac cycle, and that apparent motion illusions are eliminated. The choice of the imaging slice thickness has to be carefully considered to ensure inclusion of the tagged slice throughout the cardiac cycle, and at the same time to avoid unnecessary thickness that would only add noise to the image.
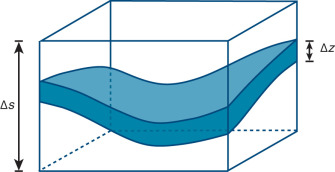
Typically, double oblique short-axis sections of the myocardium are tagged with a slice thickness of 6 to 8 mm. Subsequently, 16 to 20 sequential heart phase images are acquired with a temporal resolution (Δ t ) of about 35 ms. With this high temporal resolution, rapid motion components, such as diastolic untwisting, can be identified readily. Because the ratio of wanted to unwanted signal components must be optimized, the thickness of the imaged volume (Δ s ; see Fig. 22.2 ) must be reduced to a minimum. Therefore it depends on the level of the tagged slice with respect to its level on the long axis. For basal LV images, where a long-axis contraction of more than 20 mm may be expected for the lateral right ventricular (RV) free wall, a slice thickness of 30 mm is typically chosen. For midventricle slices, a slice thickness of 25 mm is appropriate, and at the apex, a slice thickness of 20 mm is used because of reduced through-plane motion. For suppression of breathing-induced motion artifacts, a repetitive breath – holding scheme or single breath-hold techniques can be applied. Furthermore, for high-resolution CSPAMM tagging, the two SPAMM acquisitions could be acquired with a 90-degree phase shift between them before constructing the CSPAMM images.
Considering the location of the relevant tagging information in k -space, a reduced k -space acquisition scheme can be applied. Hereby, two sets of orthogonally line tagged images are acquired. Subsequent combination of these acquisitions results in grid-tagged images ( Figs. 22.3 and 22.4 ). With this method, acquisition time is significantly reduced and image resolution perpendicular to the line tags is not affected. Fig. 22.3 shows 20 heart phase images with a temporal resolution of 37 ms acquired at an apical slice of a healthy subject. The grid structure remains visible, with a high contrast up to the last acquisition in late diastole (>700 ms). No fading of the tags is seen in the images. Therefore the method is well suited for the quantification of diastolic heart wall motion.
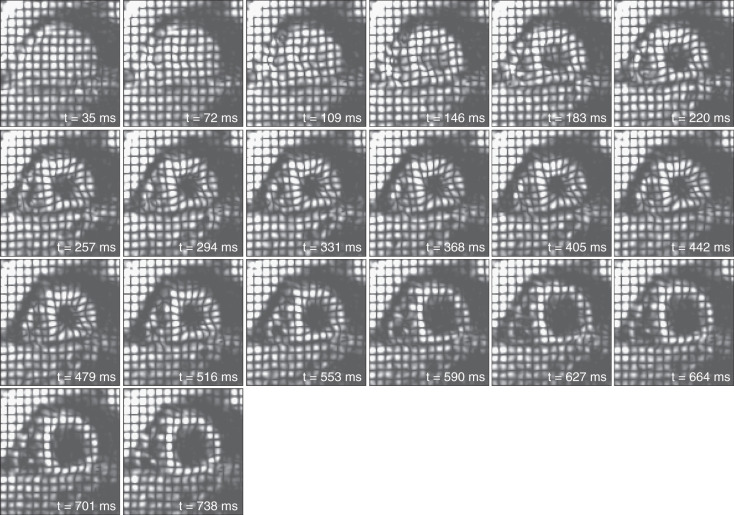
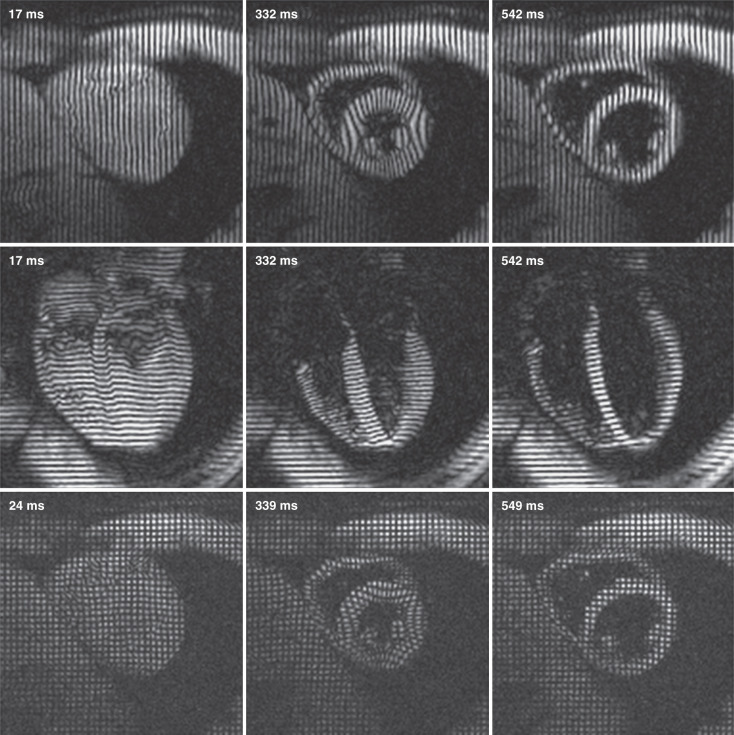
Data Acquisition Trajectory in Complementary Spatial Modulation of Magnetization
Although traditionally segmented k -space gradient recalled echo (GRE) techniques are used for signal readout, both T1 relaxation and serial RF excitations for imaging are responsible for the fading of the tags. T1 can only be increased by going to a higher magnetic field strength, and the number of RF excitations can be reduced by using alternative imaging sequences with fewer RF excitations. Hereby, echo planar imaging (EPI) (see Fig. 22.3 ) and, more recently, spiral imaging proved to be very valuable alternatives (see Fig. 22.4 ).
Spiral imaging provides a time-efficient k -space coverage, which makes it a very powerful alternative to EPI for signal readout of the tagged myocardium. Because a small number of excitation RF pulses is needed in spiral imaging, the tagging pattern persists longer, leading to improved tagging contrast. Furthermore, spiral imaging allows for efficient coverage of the k -space in a short amount of time, leading to imaging at a very high temporal resolution. Finally, the short echo time (TE) of spiral acquisition allows for minimizing flow and motion artifacts. Ryf et al. combined CSPAMM with interleaved spiral imaging, which allowed for acquiring high spatial resolution (4-mm tag separation) or high temporal resolution (77 frames per second) grid-tagged images in a single breath-hold (see Fig. 22.4 ).
Together with EPI and spiral readouts, the use of bSSFP was exploited by Herzka et al., who found that bSSFP leads to an improved tagging contrast compared with the more conventional GRE imaging. In another study, Zwanenburg et al. combined CSPAMM with bSSFP imaging for a single breath-hold scan. In this approach, the steady-state magnetization is stored in the longitudinal direction using an α /2 flip-back RF pulse immediately before tagging preparation. Imaging proceeds normally, although excitation is achieved using a series of the linearly increasing start-up angles (LISA) technique to minimize off-resonance artifacts. Ibrahim et al. developed a technique for improving the CSPAMM tagging contrast in bSSFP cine images by optimizing the RF excitation angles to compensate for tags fading during the cardiac cycle, similar to Fischer’s approach for the gradient echo CSPAMM-tagged cine images.
With the advent of parallel and 3D imaging, 3D assessment of myocardial motion based on 3D CSPAMM lattice tagging was reported by Ryf et al. Fig. 22.5 shows an isosurface-rendered image based on 3D CSPAMM imaging. Simultaneously, the same authors proposed an extension to a previously reported analysis procedure that enables relatively time-efficient analysis and quantification of both systolic and diastolic motion of the heart. However, the acquisition times were lengthy and only practical in coached breathing patterns in well-trained subjects.
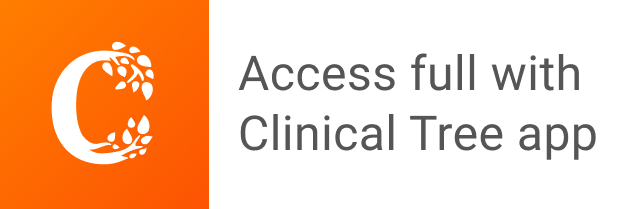