Nuclide
Half-life
Nuclear reaction
Decay mode
β± mean (KeV)
Target material
Product
Cyclotron-produced PET radionuclides
18F
109.8 m
18O(p,n)18F
β+ (96.7 %)
EC(3.3 %)
249.8
H2 18O
18F−
20Ne(d,α)18F
18O(p,n)18F
Ne/F2
18O2
18F2
11C
20.33 m
14N(p,α)11C
β+ (99.77 %)
EC(0,23 %)
386
N2 + O2
11CO2
13N
9.96 m
16O(p,α)13N
β+ (99.8 %)
EC(0.2 %)
492
H2O
13NH3
15O
122.24 s
14N(d,n)11C
β+ (99.9 %)
EC(0.1 %)
735
N2 + O2
15O2
60Cu
23.7 m
60Ni(p,n)60Cu
β+ (93 %)
EC(7 %)
970
60Ni
60Cu
61Cu
3.33 h
61Ni(p,n)61Cu
β+ (61 %)
EC(39 %)
500
61Ni
61Cu
64Cu
12.7 h
64Ni(p,n)64Cu
β+ (17.6 %)
EC(43.9 %)
β−(38.5 %)
278
190
64Ni
64Cu
86Y
14.74 h
86Sr(p,n)86Y
β+ (31.9 %)
EC(68.1 %)
660
SrCO3
86Y
89Zr
78.41 h
89Y(p,n)89Zr
β+ (22.74 %)
EC(77.26 %)
396
Natural 89Y
89Zr
124I
4.18 days
124Te(p,n)124I
β+(22.7 %)
EC(77.3 %)
820
124TeO
124I2
Generator-produced PET radionuclides
62Cu
9.67 m
62Zn/62Cu generator
β+ (97.83 %)
EC (2.17 %)
1319
82Rb
1.27 m
82Sr/82Rb generator
β+ (95.43 %)
1472
68Ga
67.71 m
68Ge/68Ga generator
β+ (88.91 %)
EC(11.09 %)
829.5
4.3 Radiolabelling with Fluorine-18
18F is the most often used radionuclide for diagnostic PET imaging since the decay properties of 18F provide significant advantages. Among the routinely produced positron emitters, the relatively longer half-life of 18F (T½ = 109.8 min) poses less constraints on synthesis time and permits longer imaging protocols to investigate processes of slower tracer kinetic up to about 6 h.
Moreover, the relatively longer half-life of 18F also permits the distribution of 18F radiopharmaceuticals to clinical centres that can be reached within a few hours of transport. In recent years, however, there has been a huge increase in the number of biologically active fluoro-organic drugs; the reason for this is directly due to the beneficial effects of simple substitution of an H atom by an F atom on the physical and/or biological properties of the molecule.
Tagging a molecule with 18F in place of a hydrogen atom often does not change its size or shape, and generally metabolically stable compounds are obtained. The unknown effects of introducing an “unnatural” fluorine atom, however, render an analogous compound with potentially changed physicochemical properties and with possibly altered biochemical, pharmacological and toxicological features. This necessitates a careful evaluation of new 18F-labelled compounds with respect to their anticipated use if they are not identical with drugs of known pharmacology [12].
With a few exceptions, radiofluorinations can be classified as either electrophilic or nucleophilic. The electrophilic reactions mainly use molecular fluorine (18F2) of moderately low specific radioactivity, or reagents prepared from it, and include additions to alkenes, reactions with carbanions and especially fluorodehydrogenation and fluorodemetallation. The nucleophilic reactions usually involve no-carrier-added (high specific radioactivity) fluoride (18F−) as its K18F-K222 complex and include SN2-type substitutions in the aliphatic series and SNAr-type substitutions in the aromatic and heteroaromatic series.
4.3.1 Electrophilic Fluorination
There are two major processes for 18F-electrophile production:
- 1.
Historical method consists in using the 20Ne(d,α)18F nuclear reaction [13, 14], where the target gas consists of natural abundance Ne containing 0.1–2 % F2 as carrier. In this system, the carrier fluorine exchanges with 18F produced by the nuclear reaction to yield 18F-19F molecules. Because of the large excess of 19F2 molecules present, the resulting specific activity is very low.
Recovery from this target system is rather slow, ranging from about 50–70 % depending upon conditions such as beam current, length of irradiation and correlates with the carrier concentration. 18F2 gas produced in the cyclotron can be directly used for electrophilic fluorination or alternatively be used as a progenitor of other fluorination reagents.
- 2.
The two bombardment method, using 18O(p,n)18F nuclear reaction and O2 gas for production of elemental fluorine 18F2 [15], offers the opportunity to produce larger quantities, however, at the expense of being more complicated.
In this approach, the first bombardment is done on passivated nickel target charged with >95 % enriched O2 and irradiated with 10 MeV protons to give 18F. 18F sticks to the target walls, while 18O2 is recovered. Refilling the target with a noble gas (Ne or Kr)/19F2 mixture and a second irradiation allows radiolitically induced isotopic exchange reactions between the adsorbed 18F and the molecular 19F2 to generate the 18F2. Specific activity is low and can be modulated by decreasing the 19F2 concentration in the mixture which unfortunately leads to a decrease of 18F2 yield.
Fluoride is a violently reactive gas that erratically reacts with organic molecules to give poor regioselectivity and mixtures of products that result from the addition across the double bond [16]. Considerable steps usually need to be taken to control the very reactive 18F2 species. The use of fluorine diluted with an inert gas gives a more controllable reagent that can react selectively with organic compounds.
Another alternative for the use of the reactive 18F2 electrophile is to convert it to the less reactive electrophilic moiety, acetyl hypofluorite (AcOF) [16, 17]. This method can be applicative for a direct labelling of small molecules [18] or peptides [17].
Other derivatives that have been used as electrophilic fluorinating reagents are 18F Fluoropyridones [19, 20] and 18F-fluoro-N sulfonamides [21]. These reagents can be used to fluorinate electron-rich substrates (such as alkenes and aryl compounds) by either direct electrophilic substitution or by demetallation reactions using organometallic reagents such as organomercury and organotin reagents. The widest use of 18F-electrophile in clinical radiopharmacy is represented by the synthesis of 18F-DOPA by regioselective, electrophilic fluorodestannylation reaction [22, 23] (Fig. 4.1).


Fig. 4.1
Synthesis of 6-18F-fluoro-L-DOPA
18F2 gas with much higher specific activity can be produced with a “post-target” method developed by Bergman and Solin [24]. This could lead to three orders of magnitude higher improved specific activity of the tracer, but it is extremely difficult to implement in clinical radiopharmacy.
In conclusion, because of the relatively low specific activity caused by the carrier-added method of 18F2 production and the poor specificity of labelling with electrophilic reagents, electrophilic 18F-fluorinations are less favoured nowadays, and the general trend is to move to nucleophilic substitution reactions.
4.3.2 Nucleophilic Fluorination
Nucleophilic 18F-fluorination reactions are routinely used to efficiently produce some of the most important PET radiotracers: virtually all 18F-labelled radiopharmaceuticals used in clinical practice are obtained by this synthetic approach. Nucleophilic 18F− is commonly produced by the nuclear reaction 18O(p,n) 18F from enriched H2 18O. The present technology for the production of 18F− consists of irradiating a small volume of enriched 18O-H2O in a metal target with protons of energies from near threshold (approximately 3 MeV) up to energy of the cyclotron, although energies above 13 MeV add little to yield while increasing the heat load on the target. Typical beam currents for research are on the order of 20–40 μA while beam currents for commercial production facilities are in the 60–100 μA range.
The new niobium target has proven to be a low maintenance target with reliable production and a good quality of 18F−. Water targets in general can produce higher specific activity 18F− which is mandatory to achieve high specific activity tracers. Specific activities of 185 GBq/μmole or more (theoretical 63 TBq/μmol) have been achieved in routine production [25]. The choice of materials and careful handling are necessary to maintain high specific activity in the final product since stable fluorine can be found in many substances [26].
18F− from the target is then trapped on an ion-exchange column which allows the recovery of H2 18O. The trapped 18F− is then eluted from the ion-exchange resin using potassium carbonate in a water/acetonitrile solution. The aqueous 18F− obtained is, however, a poor nucleophile because of its high degree of solvation. The addition of the phase-transfer reagent kryptofix-222 (K222), followed by the removal of water has proven to be crucial in improving the reactivity of the 18F fluoride ion for nucleophilic substitution reactions. The cryptand K222 forms a strong complex with the potassium cation (Fig. 4.2) and leaves the 18F− fluoride ion exposed (“naked”) and highly nucleophilic when dissolved in a polar non-protic solvent such as DMF, DMSO, or acetonitrile.
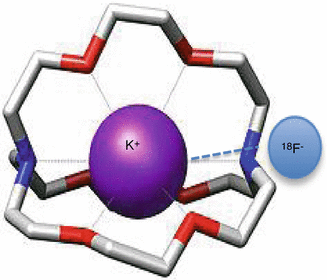
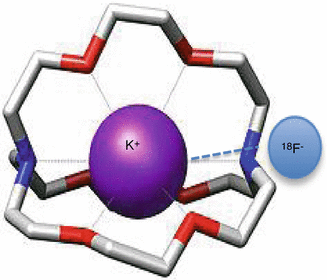
Fig. 4.2
Complexation of a potassium ion (purple) by the cryptand kryptofix-222 (K222); light blue: fluoride ion
Tetrabutylammonium (TBA) is a phase transfer catalyst alternative to K222. Comparisons between the reactivity of the two catalysts seem to support the hypothesis that TBA fluoride gives greater yields of fluorinated products in short reaction times (<10 min) [27]. Conversely K222 could cope to metallic impurities from target better than tetraalkylammonium complexes [28].
In addition to 18F-fluoride activation, the reacting precursor molecule is required to have a suitable leaving group and, in the case of aromatic rings, be suitably activated. In contrast to the wide variety of electrophilic reagents that have been developed and used with varying success, there is only one nucleophilic fluorinating reagent: fluoride ion. Nucleophilic fluorination can be performed both on aliphatic (SN2) and aromatic compounds (SNAr).
4.3.2.1 Aliphatic Nucleophilic Fluorination
Nucleophilic displacement that is usually used for aliphatic fluorination reactions involves the SN2 substitution of 18F ion and alkyl substrate containing good leaving groups such as halogens or sulfonic ester. Unlike aromatic substitution reactions, activating groups are not required.
Sulfonates are more reactive than halogens as a leaving group. There is a variety of sulfonate leaving groups available for aliphatic nucleophilic fluorination, such as p-toluenesulfonate (tosylate), methanesulfonate (mesylate), trifluoromethanesulfonate (triflate) and p-nitrosulfonate (nosylate). Among the sulfonates, reactivity increases from tosylate to mesylate and nosylate and to triflate that is the most reactive group for 18F-labelling. Nevertheless, due to its high reactivity, triflate compounds may be unstable during the fluorination reaction temperatures; they cannot tolerate any water and are subject to side reaction such as elimination.
Depending on the stability of the precursor for labelling, the reactivity and simplicity of the incorporation, aliphatic fluorination can be formed by either direct labelling [2, 29] or formation of 18F-fluoroalkyl agents [30, 31]. The main drawback of direct labelling method is the need to protect any potentially competing sites of nucleophilic attack in the molecule (principally acid, alcohol or amine groups), thus resulting in additional synthesis and purification steps.
A good example of aliphatic nucleophilic 18F substitution is the synthesis of 18F-FDG [2] in which the acetyl-protected sugar tetra-O-acetyl-2-triflate-β-mannose is used during the direct 18F-fluorination step. A deprotection of the ester groups completes the synthesis of 18F-FDG. The synthesis of 18F-FDG is now fully automated and can be achieved in approximately 30 min with radiochemical yields greater than 70 % (Fig. 4.3). A wide offer of synthesis modules, also equipped with disposable cassette that includes all the reagents, are available on the market.
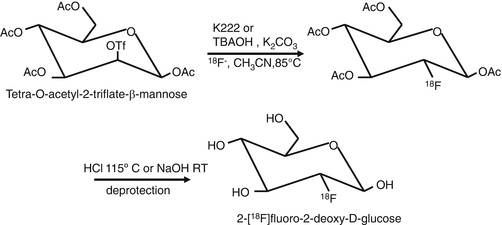
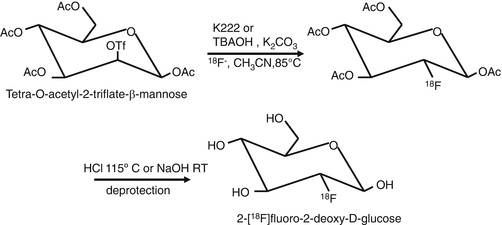
Fig. 4.3
Synthesis of 18F-fluoro-2-deoxy-D-glucose (18F-FDG)
Besides 18F-FDG, many radiopharmaceuticals have been synthesised by SN2 fluorination reactions involving aliphatic substitution such as 18F-fluoro-3-deoxy-L-thymidine (18F-FLT) [32, 33], 18F-fluoroestradiol (18F-FES) [34, 35], 18F-fluoromisonidazole (18F-FMISO) [36] and anti-1-amino-3-18F-fluorocyclobutane-1-carboxylic acid (18F-FACBC) [37], a promising tracer for staging prostate carcinoma.
Even if protic solvents, such as alcohols, are generally not used for nucleophilic substitution reactions because of their ability to solvate the nucleophile and retard its reactivity, the use of ionic solvents, such as tertiary alcohols, as a reaction media for the nucleophilic fluorination with alkali metal fluoride has been described [38, 39]. The protic medium is reported to suppress the formation of by-products and increase the rate of nucleophilic fluorination. An example is the significant improvement in the synthesis yield of 18F-FLT [40] compared to previously described methods using 18F-KF/K222 labelling procedure in aprotic solvents (Fig. 4.4).
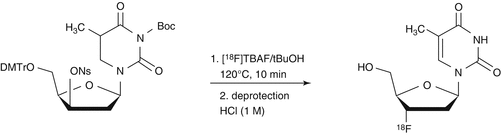
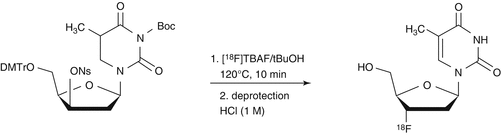
Fig. 4.4
Synthesis of 18F-FLT by reaction in tert-butyl alcohol of the protected using [18F]TBAF as phase transfer catalyst
18F-fluoroalkyl agents are synthesised by SN2 reaction of 18F-fluoride with dihalo or disulfonate alkyl starting materials with no-carrier-added fluoride ion in the presence of K2CO3/K222 complex or tetrabutylammonium hydroxide in organic solvents such as acetonitrile, o-dichlorobenzene or tetrahydrofuran [30, 31]. 18F-fluoroalkylating agents with methyl, ethyl and propyl carbon backbones together with suitable leaving groups for reaction with nucleophilic species have been prepared (Fig. 4.5).


Fig. 4.5
Synthesis of a simple 18F-fluoroaliphatic agents
The large excess of the alkyl starting material compared to the 18F− allows exclusive formation of the mono 18F-fluoroalkyl halide or sulfonate. After the formation of 18F-fluoroalkyl agent, it can be used for the second alkylation reaction. 18F-fluoroalkyl agents can be purified using gas chromatography separation or distillation from the reaction mixture into disposable C18 cartridges [31]. Purification of 18F-fluoroalkyl agent before the next alkylation reaction provides more chemically and radiochemically pure product and eliminates non-volatile impurities, therefore increasing the radiochemical yield of the second alkylation reaction.
The most known aliphatic substitution reaction using 18F-fluoroalkyl agents is the radiosynthesis of 18F-fluorocholine, in which dibromomethane is fluorinated to generate 18F-fluorobromomethane, which reacts with dimethylethanolamine to produce 18F-fluorocholine [41]. In some cases, 18F-fluorobromomethane can be converted to the more reactive synthon 18F-fluoromethyltriflate, which would react more efficiently with 2-dimethylethanolamine to give 18F-fluorocholine [31].
4.3.2.2 Aromatic Nucleophilic Fluorination
Introduction of no-carrier-added 18F into aromatic ring is mostly limited to substitution on activated arenes. The presence of “activating” electron-withdrawing groups, such as cyano, trifluoromethyl, aldehydes, ketones and nitro, in the ortho and para positions on the aromatic ring decreases the electron density allowing for a sufficient activation for the nucleophilic substitution [16]. The leaving group most widely used in aromatic nucleophilic fluorination are nitro, quaternary trimethylammonium, alogens and sulphonates such as tosylate, mesylate and triflate. Due to low specific activity of the final product, isotopic exchange, 19F-fluoride to 18F-fluoride, is not used.
Direct aromatic nucleophilic fluorination has been used for obtaining high radiochemical yields and specific activities of 18F-labelled compounds by a simple one-pot method [42]; however, not all the precursors for the labelling can handle high temperature and basic conditions of the fluorination; they may decompose during radiosynthesis. More often, the labelled compounds can be obtained by milder indirect 18F-labelling methods. An example is the use of small 18F-labelled reactive precursors bearing a reactive functional group that can form part of the intrinsic structure of the molecule or act as a “prosthetic label” to the molecule of interest such as proteins or other biomolecules. These 18F-fluoroaromatic groups can be used as 18F-precursor molecules by reacting rapidly and under mild conditions after the initial direct 18F-fluorination step. A range of 18F-fluoroaromatic precursors is shown in Fig. 4.6.
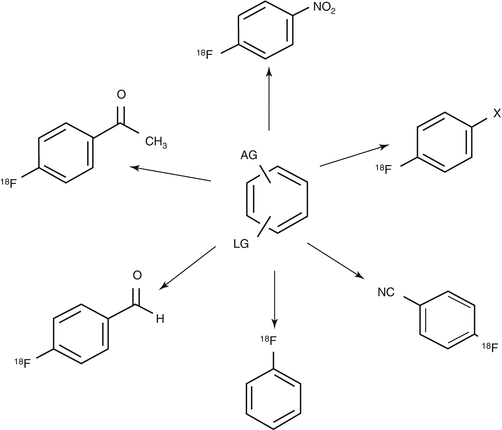
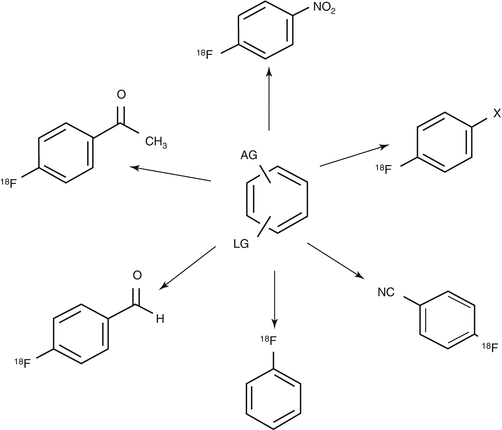
Fig. 4.6
18F-fluoroaromatic precursors synthesised by direct nucleophilic 18F-substitution (AG activating group, LG leaving group, X I or Br)
Nitrobenzene derivatives and 18F-fluorobenzaldehydes are the most widely used precursors in the preparation of simple 18F-fluoroaromatic compounds. The nitro group is both an activating group (in the ortho or para positions) and a leaving group under the right conditions. The strongly electron withdrawing nature of the nitrile group provide a high radiochemical yield of 18F-fluorobenzonitrile precursors; moreover, the nitrile group can further be transformed into reactive groups such as N-(4-18F-fluorobenzyl)-2-bromoacetamide for the 18F-labelling of peptides and oligonucleotides (see Sect. 4.3.3) [43].
The increased use and versatility of palladium-catalysed cross-coupling reactions in organic chemistry have had an important effect in the field of radiochemistry. The new chemical methods hold the unrealised potential of changing radiotracer design and development since the synthesis of relatively simple 18F-fluoroaryl precursors has, until recently, been surprisingly quite problematic. Recently it has been described the process of the successful translation of a modern Pd-mediated fluorination reaction and the application to PET imaging. Transformation of 18F-fluoride into an electrophilic fluorination reagent provides access to 18F-aryl bonds that would be challenging to synthesise via conventional radiochemistry methods [44].
Aromatic nucleophilic substitution can also be done using diaryliodonium salts and, without the need of electron withdrawing groups, fluoride can be introduced into the arene. The introduction of 18F-fluoride into dihomoaryliodonium salts precursor gives 18F-fluoroarenes and the corresponding iodoarenes [45] and represents an extremely useful alternative for the synthesis of a range of simple 18F-fluoroaromatic compounds in good radiochemical yields and in short reaction times that would be otherwise unobtainable by traditional methods. Very recently, it has been demonstrated that a novel synthetic approach to synthesise 18F-DOPA via nucleophilic substitution of a diaryliodonium salt precursor with 18F-fluoride [46] yielded a product with SA of three orders of magnitude higher than the product obtained by the traditional electrophilic destannylation with 18F2 with comparable biological behaviour and imaging properties in neuroendocrine tumour model [47]. The simplicity of the synthesis method, compared with the conventional electrophilic approach along with the possibility of injecting a dose three orders of magnitude lower in comparison with the conventional product, thus dramatically reducing the risk of pharmacologic effects due to the co-administration of 19F-DOPA, appears very promising.
4.3.3 18F-Labelling of Biomolecules
Biomolecules such as peptides, proteins, affibodies, antibodies and oligonucleotides can be labelled with fluoride-18 and evaluated for their potential as diagnostic imaging agents. Considering the relatively short 18F half-life, labelling biomolecules with 18F needs a careful consideration of the tracer kinetics since a fast clearance from the blood and high accumulation in target tissue should be required. Out of the biomolecules mentioned above, peptides fit these demands, with rapid clearance from the blood and high concentrations in target tissue. Moreover, the small size of peptides usually makes them relatively easy to synthesise with chemical modification, if needed, and they can often tolerate harsh chemical conditions for radiolabelling.
Larger biomolecules, such as antibodies, have slow pharmacokinetics (slow clearance from the blood) and high nonspecific binding, and they usually have lower uptake in target tissue and sometimes dependent on protein concentrations in the short time frame of the imaging.
Direct nucleophilic fluorination with 18F-fluoride is not generally appropriate with larger peptides and proteins because of the high temperatures, organic solvents and basic conditions needed to obtain a good radiochemical yield. The strategy for labelling peptides and proteins for PET studies is based on the introduction of 18F radionuclide by reaction with suitable prosthetic group under mild reaction condition. There is no general protocol for the synthesis of labelled peptides for PET, and often several labelling procedures need to be explored and optimised to find the best method for a particular peptide.
Many of these prosthetic 18F-groups have been synthesised for targeting amino, carboxylic acid, or sulfhydryl functional groups within the peptide. N-terminal primary amino groups and lysine residues in proteins or peptides have received the greatest attention.
To date, the most common 18F-prosthetic group for labelling biomolecules through the reactive amine group of lysine is the N-succinimidyl-4-18F-fluorobenzoate (18F-SFB) [48]. 18F-SFB can be synthesised in different routes, starting from different precursors, but it requires a time-consuming three-step synthesis. Recently, however, significant advances have been taken to automate its synthesis [49]. Coupling of 18F-SFB with peptides or proteins can be performed under mild pH and temperature conditions in aqueous media (pH 8–9). Acylation with 18F-SFB was shown to be a convenient labelling method in terms of in vivo stability and radiochemical yield.
4-18F-fluorobenzaldehyde (18F-FBA) has also proven to be a versatile labelling reagent that is significantly easier to prepare than 18F-SFB. Chemoselective 18F-labelling, with high radiochemical yields and under mild reaction conditions, of peptides having an amino-oxyl functional group (via the formation of an oxime group) can be achieved using 18F-FBA [50].
Another possible method involves labelling a thiol group (e.g. in cysteine) using 18F-prosthetic group maleimide (18F-maleimide) and its derivatives (18F-FBABM) [51]. The radiosynthesis of 18F-maleimide and its derivatives can also be done through the formation of other 18F-prosthetic groups such as 4-18F-fluorobenzaldehyde or 18F-FBA that could be further reacted with different maleimide precursors to give various derivatives of 18F-maleimide prosthetic groups [51]. 4-18F-fluorobenzaldehyde is capable of forming bond with hydrazino group in the biomolecule, to form hydrazone [52].
18F-glycosylation reactions of amino acids and peptides using chemoselective 18F-fluoro-glycosylated derivatives of 18F-FDG have been reported to be an effective way of introducing an 18F-label [53]. The glycosylation of biomolecules, such as peptides or proteins, has been frequently shown to improve the in vivo kinetics and stability in blood, to enhance bioavailability and BBB permeability and to accelerate the clearance of such glycoconjugates in vivo. Moreover, it has been shown by that glycosylation of peptides with subsequent radiolabelling opens the way to radiotracers with improved in vivo properties. The area of 18F-glycosylation reactions has recently and comprehensively been reviewed [54].
The reaction of 1,3-dipolar cycloaddition (Huisgen reaction), flexible 18F-labelling chemistry known as “click chemistry” and its use in radiochemistry were reported in 2006 [55] for the preparation of 18F-labelled peptide fragments. Especially the Cu(I)-catalysed variant of the Huisgen 1,3-dipolar cycloaddition of terminal alkynes and azides (Cu-catalysed azide-alkyne cycloaddition, CuAAC) offers a very powerful reaction with high specificity and excellent yields under mild conditions [56].
18F-labelled alkynes were prepared by the 18F-nucleophilic substitution reaction of an alkyne tosylate. The large stoichiometric excess of the CuI catalyst and azide compared to the 18F-alkyne results in good to excellent radiochemical yield for the conjugation step within 10 min at room temperature under basic conditions. The labelled compounds were obtained in high purity by using a simple purification method based on a C18 cartridge followed by evaporation of the eluent solvent and excess 18F-fluoroalkyne. As a result, numerous PET tracers have been synthesised using CuAAC in a widespread spectrum of structural varieties of the prosthetic group within the last decade.
In 2007, it has been reported for the first time [57] 18F-PEG derivatives as new 18F-labelled prosthetic click groups. These compounds showed a reduced volatility and increased polarity compared with other 18F-labelled prosthetic groups like 18F-FEA or 18F-fluoroalkynes. 18F-labelled PEGylated prosthetic groups have been widely employed by for labelling peptides and nanoparticles [58–60]. 18F-gluco derivatives for CuAAC-radiolabelling have been developed in order to improve the in vivo behaviour of peptides with respect to blood clearance and stability [54, 61].
However, the need of cytotoxic copper during CuAAC has led to the necessity of alternative fast and copper-free click reaction strategies for radiofluorination and additionally enabling pretargeting approaches in living systems. This has led to the development of copper-free click-labelling reactions which have been focused on derivatives of cyclooctynes and dibenzocyclooctynes [62] or on the possibility to perform Cu-free click reactions given by the inverse electron demand of the Diels-Alder cycloaddition between a cyclooctene and a tetrazine [63]. A detailed review on the development of click chemistry for 18F-labelling has been recently published [64].
The field of click cycloadditions has a major impact in 18F-labelling chemistry. Very mild reaction conditions, excellent efficiency and protection group chemistry not needed are particularly suitable for 18F-labelling especially for complex and sensitive biomolecules such as peptide, proteins and oligonucleotides.
Silicon has a high affinity for F, allowing facile introduction of 18F under mild conditions facilitating direct 18F-labelling to Si-conjugated biomolecules. In silicon-based 18F-fluoride acceptor (Si-FA) moieties, the Si atom is associated with an aromatic group, and 18F-labelling of the Si is achieved by isotopic exchange or substitution of an OH group. The 18F-Si-FA is then conjugated to the biomolecule. Since the side groups attached to the Si atom affect the stability of the 18F-Si bond in the Si-FA moiety to hydrolysis, it has been demonstrated that tert-butyl groups dramatically improved the stability of the 18F-Si complex in the labelling of peptide moieties [65, 66].
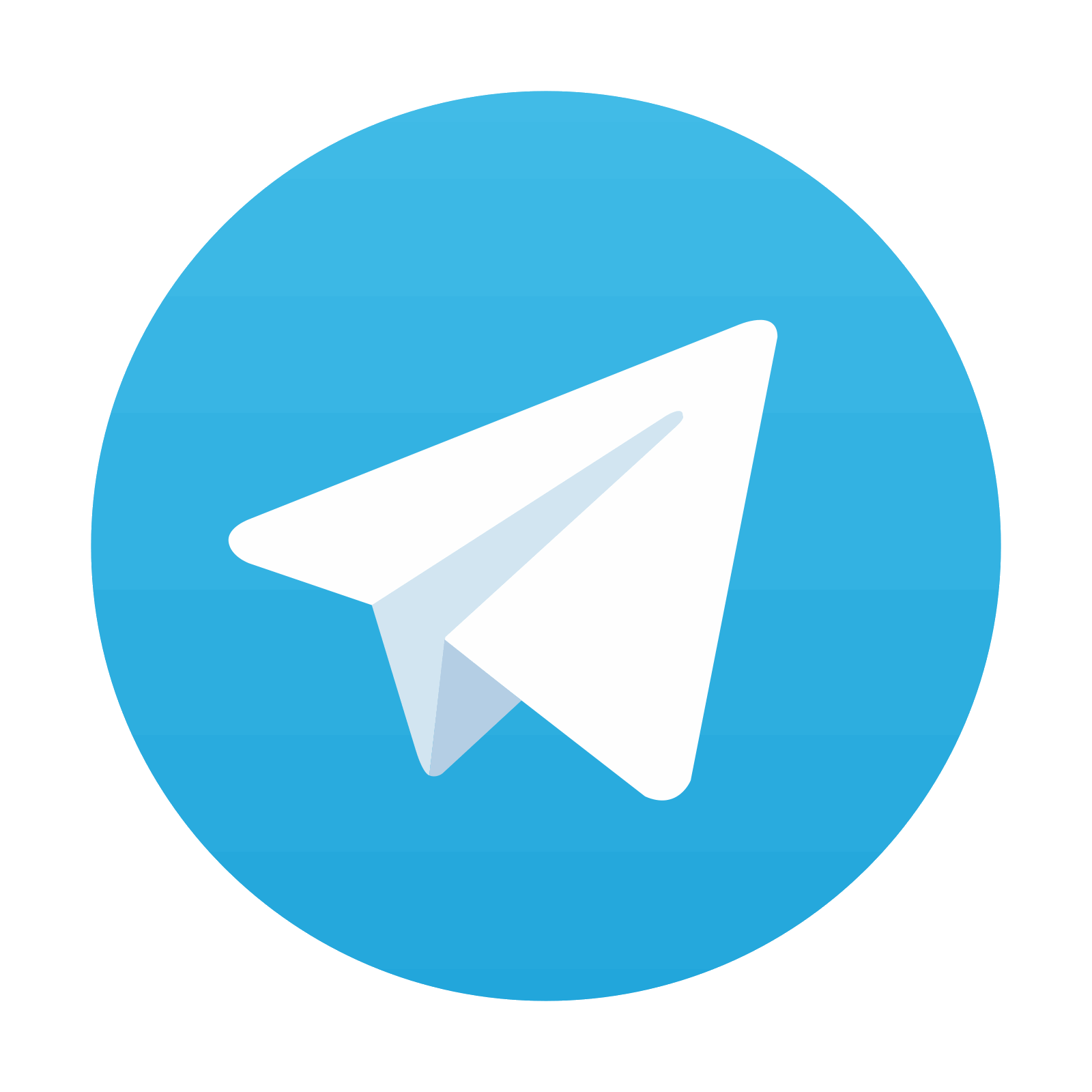
Stay updated, free articles. Join our Telegram channel
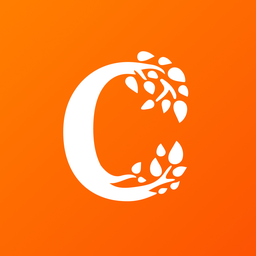
Full access? Get Clinical Tree
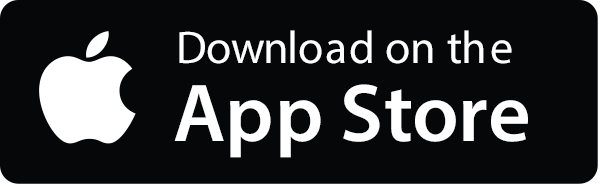
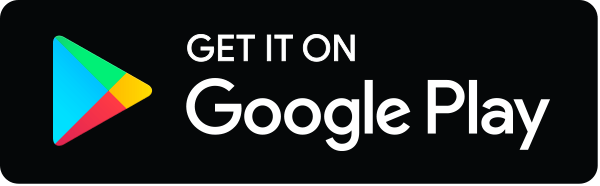