Fig. 12.1
Ultrasound-assisted opening of blood-brain barrier in a rat model. One megahertz focused ultrasound (IGT, 1 Hz, 20 ms pulses, 1–2 min) treatment of rat brain following intravenous administration of decafluorobutane microbubbles (DSPC/PEG stearate shell). Imaging of focal areas 1–6 observed as T1 MRI contrast with Gd-DTPA extravasation and accumulation (UVA Molecular Imaging Center (7 T MRI Clinscan, Bruker/Siemens) © Max Wintermark, 2014, with permission)
This approach has been expanded from BBB opening to enhancing delivery across the vessel wall in other tissues, e.g., hindleg muscle and tumor (Bohmer et al. 2010), from dyes to viruses (Muller et al. 2008); with multiple uses for improvement of tissue delivery of a wide range of drugs. Even more exciting is the ability to enhance tissue delivery specifically in the ultrasound treated areas, while minimizing drug deposition in the normal non-diseased tissues. Exact mechanism of this prolonged vascular permeability enhancement is still debated: from the transient gap junction degradation (Alonso et al. 2010) to enhancement of endocytosis (De Cock et al. 2015) and transcytosis (Sheikov et al. 2004).
An exciting example of an early stage clinical trial for co-administration approach has been reported already (Kotopoulis et al. 2013), in a pancreatic cancer primary tumor setting. Pancreatic cancer is known to have generally low vascularity (Rodallec et al. 2006), so drug delivery from the bloodstream into the tumor mass is restricted. Drug of choice, gemcitabine, stays in the bloodstream for at least an hour following intravenous administration (Gemzar 1996); thus, when ultrasound imaging of pancreas and the cancer nodule is performed, repeatedly, with multiple injections of SonoVue microbubbles and corresponding imaging/insonation cycles (Fig. 12.2), the vascular barrier to drug delivery into the tumor tissue is “softened”.
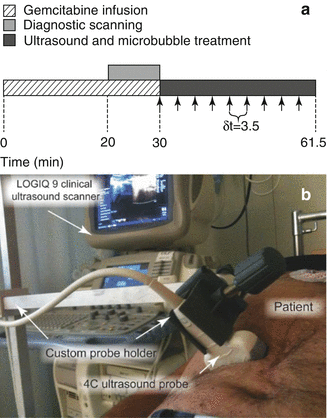
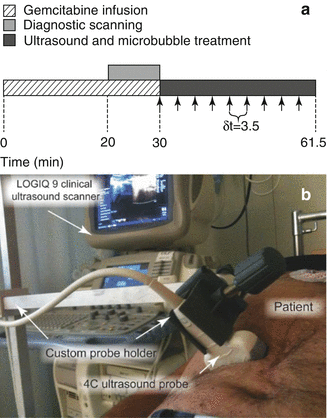
Fig. 12.2
Time frame of each chemotherapy cycle [panel (a)] and photograph of probe and custom-made probe holder during patient treatment using microbubble sonoporation for pancreatic cancer [panel (b)]. Panel (a) shows the time frame for each treatment cycle from the start of the gemcitabine infusion. Arrows indicate intravenous injection time of 0.5 ml SonoVue followed by a 5-ml intravenous injection of saline. Time between each injection (δt) is 3.5 min © American Association of Physicists in Medicine, 2013 (Reprinted with permission from Kotopoulis et al. (2013))
Consequently, recirculating drug may have much better chances to enter the tumor from the bloodstream, providing therapy; slowing down tumor growth in patients who did receive ultrasound and microbubbles in comparison with a historical cohort of the standard-of-care patients was demonstrated in this first-in-human trial (Fig. 12.3). A continued expanded study from the same group of authors may point towards an exciting evidence of life extension (Dimcevski et al., manuscript in submission).
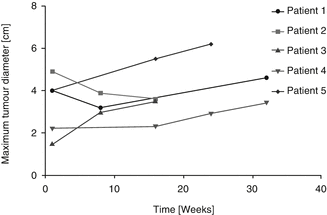
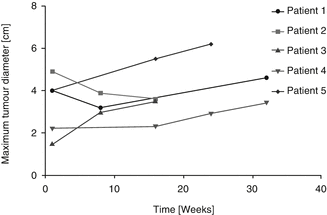
Fig. 12.3
Change in tumor diameter over time measured from CT images in patients with pancreatic malignancy © American Association of Physicists in Medicine, 2013 (Reprinted with permission from Kotopoulis et al. (2013))
12.2.3 Tissue Geometry, Mechanics and Distance as Barriers to Drug Delivery
We have already discussed above that inertial (destructive) as well as stable cavitation of microbubbles in the ultrasound field enhance delivery into the cells and across the endothelial lining. However, there are numerous other barriers that the drug may have to cross, beyond the cell membrane and endothelium. First, there is a layer of pericytes on the outside of endothelial lining, wrapping around capillaries and post-capillary venules. In larger vessels, there are layers of smooth muscle cells; large vessels are quite thick, with distinguished layers of mechanically robust intima, media, and adventitia. Mechanical properties of the vessel wall may limit drug passage, even after endothelial lining “softening” by ultrasound, so in large vessels one may prefer to deliver the drug just to the intima, e.g., an anti-restenosis drug (Phillips et al. 2011). If the drug should be delivered to the bulk interstitial space, perhaps ultrasound-assisted delivery across the vessel wall at the capillary or post-capillary level may be more efficient. Next barrier is the tissue itself: the space from the vessel into the bulk of tissue may extend beyond tens of microns which makes it difficult for the drug molecule to penetrate by diffusion through the crowded interstitial space. Specialized drug or nucleic acid carrier systems can be then applied – e.g., highly pegylated nanoparticles that can penetrate through the tissue rapidly, as proposed by Hanes et al. (Nance et al. 2014b). Combination of focused ultrasound and intravenous microbubbles with PEG-PEI-plasmid resulted in an excellent delivery and transfection efficacy (Burke et al. 2012) as well as deep tissue penetration (Nance et al. 2014a). Acoustic radiation force action on microbubbles may also be of assistance: ultrasound field can improve microbubble delivery to the target surface from the bulk of blood vessels, reducing the required microbubble dose (Dayton et al. 1999); ultrasound-induced microstreaming may also assist drug delivery.
12.3 Drug-Carrying Sonosensitive Particles
12.3.1 Drug-Loaded Nano/Microbubbles
The driving force of drug delivery by sonoporation with nano/microbubbles is stable (or inertial) cavitation, which leads to release of sequestered drug from the particle, as well as increase of permeability of adjacent cell membranes. For efficient delivery, the drug should be present while a bubble is acting on the cell membrane. This is where drug-loaded bubbles may prove beneficial.
Recently, numerous research groups have been developing drug-carrying bubbles, including drug complexes with microbubbles, nanobubbles, nanodropletes, liposomes, emulsions and micelles. The range of tested drugs is quite broad, from small to large molecules, from inorganic to organic to biomolecules. Various types of drugs have been already associated with bubbles, from anti-cancer agents (Gao et al. 2008; Rapoport et al. 2009a), small interfering RNA (Negishi et al. 2008; Endo-Takahashi et al. 2012), micro-RNA (Hatakeyama et al. 2014; Endo-Takahashi et al. 2014), antisense oligonucleotides (Koebis et al. 2013), plasmid DNA (Endo-Takahashi et al. 2013), to proteins (Bioley et al. 2012a, b, 2013). This chapter section focuses on the advances of drug-loaded ultrasound-sensitive particles, discusses their formulation, properties, issues and prospects toward future clinical use.
12.3.2 Formulations and Properties
Formulations are usually based on a perfluorocarbon (PFC) core (gas or liquid), stabilized by a shell. PFCs are hydrophobic. To suspend and stabilize them in physiological media such as saline or isotonic sugar solution (e.g., 5 % glucose) we need to use the shells made of amphipathic material, which could be a protein, surfactant and/or lipid. In the commercially available microbubbles, albumin or lipids are utilized as the shell stabilizers. These molecules have hydrophobic and hydrophilic groups in their structure. Hydrophobic groups (e.g., exposed core of denatured albumin) face the PFC (gas or liquid) core, and hydrophilic groups face the aqueous phase to inhibit particle aggregation and fusion. Gas-filled particles with amphipathic polymer shell have also been studied. In the early phase of drug loading experiments, hydrophobic drugs were selected and loaded in the bubble shell via hydrophobic interaction. Bubble shell surface charge can be modified to attach the drugs (mostly nucleic acids) via electrostatic interaction. As an option to improve drug load, the drug could be covalently attached to the microbubble shell. Lately, newer types of bubbles have been developed, in which nanobubble was encapsulated inside liposomes (Suzuki et al. 2007; Javadi et al. 2012). These liposomal bubbles may co-encapsulate the drug in the inner aqueous phase of liposomes. In this section, we introduce the formulations of drug-loaded bubbles.
12.3.2.1 Drug-Particle Formulations Via Hydrophobic Interaction (Non-covalent Binding)
During the past decade many research groups have reported drug-loaded microbubble formulations. Initially, a hydrophobic anti-tumor agent, paclitaxel, was tested for its ability to associate and retain with the microbubbles shell (Unger et al. 1998; Tartis et al. 2006). Hydrophobic drugs are simply mixed with microbubble shell material and associate with the hydrophobic layer of the shell (Scheme Fig. 12.4a). These drugs are released, possibly in combination with shell fragments, when ultrasound is applied and bubbles are destroyed. Just as traditional ultrasound contrast microbubbles, drug-loaded microbubbles may be visualized with ultrasound imaging, which can provide additional information in the image-guided drug delivery applications.
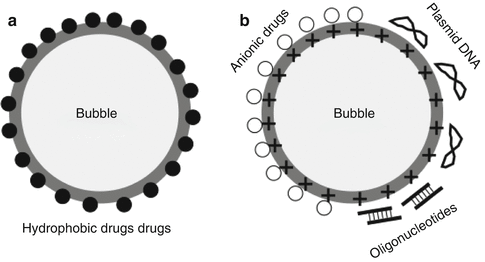
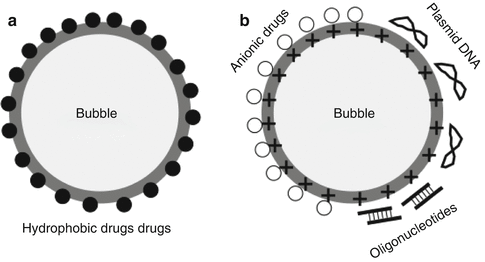
Fig. 12.4
Structure of drug-loaded microbubbles. (a) Drug retained within the bubble shell via hydrophobic interaction. (b) Drug and nucleic acids attached to the bubble shell via electrostatic interaction
The endothelial gap junction pores in the normal tissue vasculature are less than 7 nm (Rapoport et al. 2009b), which may limit delivery of nanoparticles and biopharmaceuticals out of the vasculature. In the tumor tissues blood vessels are defective and leaky. In addition, lymphatic drainage in tumor tissue is poor as compared to normal tissues (Matsumura and Maeda 1986). These features allow so-called “passive targeting” to achieve drug and gene delivery and accumulation in the tumors. This phenomenon is known as the enhanced permeability and retention (EPR) effect (Matsumura and Maeda 1986). Microbubbles, with their micrometer dimensions, are optimal as intravascular imaging agents, but their size is too large to penetrate through the vascular endothelial lining in tumors: typically, particles have to be significantly less than 1 μm in diameter to extravasate into the tumor interstitial space (Yuan et al. 1995). Therefore, to deliver drugs to the tissues outside of the vascular bed, nanobubbles (with gas core) and nanodroplets (with liquid core), which are less than 1 μm have been developed. These are generally prepared with sonication. In brief, aqueous solution or nano-dispersion (e.g., micelles) of shell material is sonicated in the presence of perfluorocarbon gas or liquid. Hydrophobic drugs can be loaded in the shell of these particles by hydrophobic interaction. Nanodroplets are usually prepared from liquid perfluoropentane and perfluorohexane and stabilized with a polymer coat or a lipid monolayer shell (Rapoport et al. 2009a, 2011; Mohan and Rapoport 2010). With submicron size, nanodroplets can exit tumor vasculature after intravenous injection and accumulate in the tumor interstitium via EPR mechanism. The boiling points of perfluoropentane and perfluorohexane, which are utilized as liquid core in nanodroplet, are 29 °C and 56 °C, respectively. Due to surface tension, perfluoropentane nanodroplets may remain liquid even at 37 °C, in “superheated” state. However, they can rapidly convert (shift) to gas bubbles in response to ultrasound (Fig. 12.5).
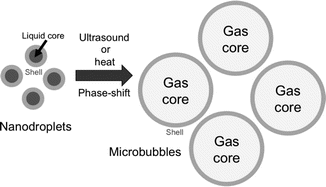
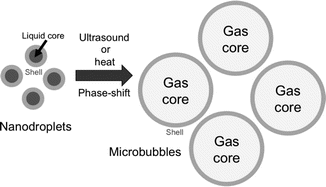
Fig. 12.5
Structure of nanodroplets and their phase-shift conversion to microbubbles. Nanodroplets contain liquid perfluorocarbon core (e.g., C5F12), stabilized with a lipid or polymer shell. Stimulation with ultrasound irradiation and/or heating results in conversion of the liquid core phase to gas phase, with microbubble formation
Therefore, these particles are sometimes called phase-shift nanodroplets or nanoemulsion (Marin et al. 2001). This phase shift can be induced by targeted focused ultrasound in–vivo, for example, to convert the nanodroplets that have accumulated in the tumor tissue by EPR effect after intravenous injection. As a result, drug can be released from the particles only at the site of their phase shift by ultrasound exposure. Resulting gas microbubbles can be observed by ultrasound imaging. Therefore, this system might be useful for imaging control of triggered drug delivery.
Gas-core micro- and nanobubbles and liquid-core nanodroplets usually exhibit a relatively short in-vivo lifetime due to core loss (Shiraishi et al. 2011). Lower molecular weight PFCs (usually gases) are better soluble in water and blood; PFC core is lost from the shell and particles become insensitive to ultrasound. Behavior of nanobubbles and nanodroplets under ultrasound exposure (inertial cavitation with rapid collapse) may become uncontrolled. Consequently, damage to cells and tissues might be induced if acoustic pressure is above the mandated ultrasound contrast imaging Mechanical Index (MI) limits. As a “softer” alternative, Zhang et al. (2014) developed an interesting phase shift “solid–liquid-gas” tri-phase transition particle. It is based on a natural “solid–liquid-gas” tri-phase transition medium, L-menthol as an inner core; hollow mesoporous silica is used as the outer shell. The nanoparticle can continuously generate volatile gas in a relatively mild manner, rather than the conventionally abrupt phase transition induced by boiling of superheated PFC liquid. This controllable solid–liquid-gas tri-phase transition of L-menthol can be attributed to the gradual liquid to volatile gas phase-transition at far below its boiling point (212 °C). The generation of L-menthol gas permits extended enhancement of drug delivery following a single bolus injection. In this paper, they reported prolonged blood circulation time (T1/2 = 130 min) and continuous accumulation in the tumor during 24 h period. These particles have porous structure, so they can be loaded with hydrophobic and hydrophilic drugs at the same time. Synergistic effects of high intensity focused ultrasound therapy and chemotherapy with these particles could be expected.
12.3.2.2 Electrostatic Complexes
This loading style is mostly applied for negatively charged molecules such as nucleic acids and some proteins. Microbubbles or nanobubbles with cationic shells (cationic lipids or polymers) are used. This approach has been applied for almost three decades to prepare liposome-nucleic acid complexes for lipofection. Particles are simply mixed with negatively charged molecules (e.g., plasmid DNA, or oligonucleotides) in low or moderate ionic strength media and complexes are formed by electrostatic interaction (Fig. 12.4b). Electrostatic adsorption onto the bubble shell has been shown to protect nucleic acid from degradation by nucleases, as well as enhance loading efficiency. Net positive charge of the complexes also helps attach the bubbles to cell membrane, and further improve delivery efficiency. This design was first reported by (Unger et al. 1997) who prepared positively charged microbubbles as a modification of MRX-115 microbubbles (lipid shell of that parent formulation is based on zwitterionic dipalmitoyl phosphatidylcholine). In the microbubbles intended for gene delivery, a 1:1 mixture of positively charged dipalmitoyl ethylphosphatidylcholine (DPEPC) and zwitterionic dioleoyl phosphatidylethanolamine was utilized. These microbubbles bound plasmid DNA encoding chloramphenicol acetyl transferase, and could enhance transfection of plasmid DNA into cultured cells when 1 MHz continuous wave ultrasound was applied for 5–30 s.
Many research groups have been utilizing electrostatic binding not only for plasmid DNA but also for oligonucleotides such as antisense, small interfering RNA (siRNA), microRNA, mRNA and minicircles. Various compositions of microbubbles and nanobubbles have been developed and tested as gene delivery systems in-vitro and in-vivo. To improve stability of microbubble formulations, use of longer-chain lipids with higher phase transition temperature was suggested (Christiansen et al. 2003). In that study, a mixture of cationic distearoyl trimethylammonium propane (DSTAP) and neutral distearoyl phosphatidylcholine (DSPC) and poly(ethylene glycol) stearate, with longer-chain stearoyl (C18 acyl) groups, was used for bubble preparation. The resulting shell served as an effective stabilizer for micrometer- size perfluorobutane gas core. Refrigerated storage of these microbubbles for many months in sealed vials under perfluorobutane atmosphere was reported. After complexing with plasmid, they have provided efficient transfection in the insonated skeletal muscle or myocardium in-vivo (Christiansen et al. 2003). Stability of microbubble formulations may be a major factor for gene delivery efficiency (Alter et al. 2009).
The type of cationic molecules is important for efficient transfection, as is the ratio between cationic lipids and nucleic acids. The latter is usually described as N/P ratio. N represents total number of aminogroups (nitrogen atom, positive charge) derived from lipid in bubbles; P represents total number of phosphate groups (phosphorus atom, negative charge) derived from nucleic acids. Optimization of N/P ratio is as important for sonoporation as it is for conventional gene delivery methods based on cationic molecules such as lipofection or polyplex. Takahashi et al. reported that distearoyl dimetylammonium propane (DSDAP)-based cationic liposomal nanobubbles had the highest gene delivery efficiency in comparison with other cationic lipid formulations, such as DSTAP or dimethyldioctadecylammonium bromide (DDAB) (Endo-Takahashi et al. 2013). DSDAP-based plasmid DNA-loaded nanobubbles achieved therapeutic effect in mice with hindlimb ischemia by bFGF gene transfer with ultrasound. Lately, nucleic acid delivery is focused on siRNA and microRNA, the novel gene therapy technologies. For gene silencing, siRNA molecules should be delivered into the most of target cells to inhibit expression of a desired gene. However, a simple co-injection of microbubbles and free nucleic acids results in very low transfection efficiency. Yet even sonoporation with nucleic acid-loaded bubbles would not be sufficient to achieve the desired transfection levels. To overcome this problem, Un et al. (2010a, b, 2011) developed active targeting of nucleic acid-loaded nanobubbles, mannose-modified cationic liposomal bubbles, which utilized mannose-modified PEG-lipids for macrophage targeting. In their initial reports, plasmid DNA-loaded mannose-modified liposomal bubbles provided higher transfection efficiency than non-targeted liposomal bubble (Un et al. 2010a, b). Similar mannosylated sonosensitive siRNA lipoplexes targeted to hepatic endothelium suppressed intracellular adhesion molecule-1 (ICAM-1) expression (Un et al. 2012). Moreover, this group has reported lipopolyplex bubbles, prepared as electrostatic complexes of plasmid DNA and cationic polymer with anionic liposomal nanobubbles (Kurosaki et al. 2014). These bubble lipopolyplexes could reduce cytotoxicity, aggregation with erythrocytes and inflammatory response, the usual problems of cationic gene delivery tools. There is another elegant gene delivery system. Chen et al. developed beta cell-specific gene expression system in pancreatic islets by the combination of ultrasound and plasmid DNA-loaded microbubbles (Chen et al. 2006; Chai et al. 2009). In this study, rat insulin promoter-driven human insulin plasmid DNA and lipofectamine 2000 (commercially available cationic liposomes for gene delivery) were mixed, and the electrostatic complexes were added to phospholipids suspension in the perfluoropropane gas-filled sealed vial and shaken in an amalgamator to prepare microbubbles. Following intravenous infusion of these microbubble-lipofectamine-plasmid complexes, ultrasound was directed at the pancreas to destroy microbubbles within the pancreatic microcirculation, and islet-specific transgene expression in pancreas was observed. Expression peaked at day 4 and then decayed steadily over 4 weeks following a single treatment. Later, these researchers have expanded their studies to sonoporation gene delivery regeneration therapy (Chen et al. 2007, 2012, 2014). Overall, electrostatic drug-microbubble complexes play an important role in the development of nucleic acid sonoporation delivery systems.
12.3.2.3 Covalent Coupling of Drug Substances onto Microbubbles
There are other drug loading methods, based on covalent coupling between the drug and the bubble. Covalent coupling of biomolecules to microbubbles has been assessed and optimized for targeted molecular ultrasound imaging for almost two decades, as described and reviewed elsewhere (Villanueva et al. 1998; Klibanov 2005). For therapeutic protein delivery applications (Fig. 12.6), Bioley et al. developed antigen-decorated microbubble formulations (Bioley et al. 2012a, b, 2013), and demonstrated that microbubbles can serve as an efficient antigen delivery system to promote phagocytosis of the model antigen ovalbumin (OVA) even without ultrasound exposure: in-vivo administration of OVA-loaded microbubbles in mice resulted in the induction of OVA specific antibody and T cell responses.
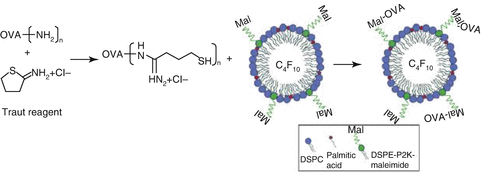
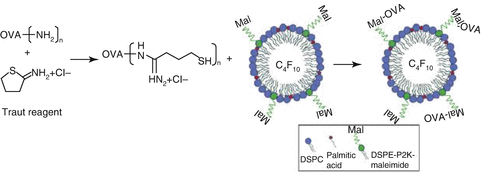
Fig. 12.6
Schematic representation of thiolated OVA linkage to microbubble via maleimide-lipid anchored to the microbubble shell. © Elsevier Ltd, 2012 (Reprinted with permission from Bioley et al. (2012b))
12.3.2.4 Particle-Decorated Microbubbles
There are specialized loading methods to protect nucleic acid from nuclease and improve per-particle loading efficiency. Positively charged liposomes, lipoplexes and polyplexes that entrapped and/or stabilize nucleic acids or drug-loaded nanoparticles, can be placed on the surface of the microbubbles, either via streptavidin-biotin bonds (Vandenbroucke et al. 2008; Yang et al. 2013), or by covalent coupling (Sirsi et al. 2012). In addition, there are reports that describe virus-coated microbubbles. Porter, Bamber et al. (Taylor et al. 2007) developed a microbubble complex with an entry-deficient retrovirus, where positively charged microbubbles carried viral particles attached electrostatically. As mentioned above, these particle-loaded microbubbles were formed by combining pre-formulated microbubbles with particles. Alternative approach is also possible: Bekeredjian et al. prepared adeno-associated virus (AAV) vector-loaded microbubbles by co-amalgamation of viral particles with lipids in water-glycerol solution, by a rapid (45 s) mechanical activation with a Capmix/Vialmix amalgamator and succeeded to develop an ultrasound gene delivery system in a cardiac setting (Muller et al. 2008). Lentacker and colleagues (Geers et al. 2011) developed an in-situ microbubble-liposome complex preparation method, where drug-loaded liposomes (Doxil) were added to glycerol:propyleneglycol:water media containing DSPE-PEG-maleimide, dipalmitoyl phosphatidylcholine (DPPC) and distearoyl-sn-glycero-3-phosphoethanolamine-N-[PDP (polyethylene glycol)-2000] (DSPE-PEG-PDP) in sealed vials with decafluorobutane headspace, followed by the same amalgamation step. This mechanical activation gives rise to C4F10 lipid-shelled microbubbles decorated with liposomes. The liposomes are attached to the microbubble shell via covalent thiol-maleimide linkages; respective DSPE lipid anchors on the termini of the conjugate are embedded in the liposome bilayer and bubble monolayer shell. Finally, it was reported that doxorubicin-liposome-microbubbles allowed ultrasound-triggered killing of melanoma cells in-vitro even at very low doses of doxorubicin (Escoffre et al. 2013). The advantage of this approach is in the small size, low price and availability of amalgamators. Ease of use and short time of the preparation ensure ability to generate sterile microbubble-particle complex at the bedside.
12.3.2.5 Liposomal Bubbles
Liposome is an intelligent drug/gene delivery tool because of its biocompatibility, flexible composition and ease of loading of various types of drugs (including nucleic acids) inside and outside of the particle. Targeting molecules can be placed on the liposome surface for selective drug delivery to receptor-carrying cells and surfaces. Recently, liposome-based nanobubbles were developed. Suzuki, Maruyama and Negishi et al. reported a sonosensitive liposome particle encapsulating a perfluoropropane nanobubble inside it (Fig. 12.7). This liposomal bubble was termed “Bubble liposome” (Suzuki et al. 2007, 2008; Kodama et al. 2010). For plasmid DNA, siRNA and miRNA delivery, they utilized cationic Bubble liposomes loaded with these nucleic acids. As an alternative siRNA-loading method, they used hydrophobic anchor, cholesterol modified siRNA (Negishi et al. 2011). Targeting of Bubble liposomes with specific ligands further enhanced gene delivery efficiency.
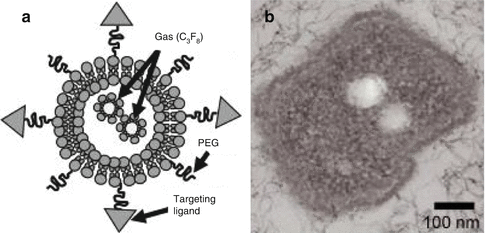
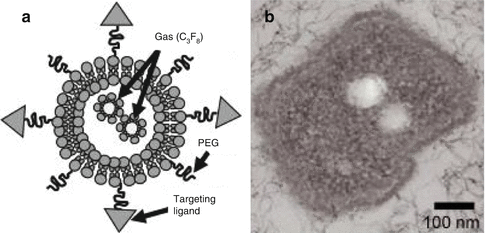
Fig. 12.7
Structure of Bubble liposome. (a) Cartoon of Bubble liposome, (b) Transmission electron microscopy of Bubble liposome. Original magnification, ×50,000. JEOL JEM2000EX operated at 100 kV. © Elsevier B.V. (Reprinted with permission from Suzuki et al. (2008))
A similar formulation with a similar structure, named “eLiposome” was developed recently by Pitt et al. (Fig. 12.8). This particle entrapped perfluoropentane and/or perfluorohexane nanodroplet inside a liposome (Javadi et al. 2012; Lattin et al. 2012a). First, nanoemulsion of perfluoropentane and/or perfluorohexane was prepared. Then nanodroplets were encapsulated in liposomes by co-sonication for the mixture of nanodroplets and liposomes, or by hydration of dry lipid film with the aqueous dispersion of nanodroplets. Finally, nanodroplet encapsulated liposome was purified with step-wise density gradient centrifugation (Javadi et al. 2012). This eLiposome particles were sensitive to ultrasound exposure: co-entrapped calcein release was demonstrated (Lattin et al. 2012b). It is suggested that ultrasound helps convert liquid fluorocarbon to gas, leading to a drastic increase of volume and rupture of the surrounding membrane. Targeted, folate modified eLiposome was developed and demonstrated targeted enhanced delivery of calcein and plasmid DNA into HeLa cells with ultrasound (Javadi et al. 2013). Likewise, folate-modified doxorubicin encapsulated eLiposome was also developed; it could effectively deliver doxorubicin into HeLa cells and provided cytotoxicity enhancement (Lin et al. 2014).
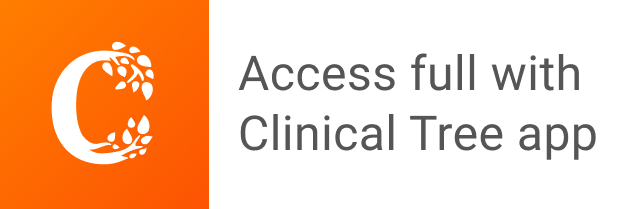