Fig. 65.1
Megavoltage dose equivalent in rads (cGy) versus number of treatment fractions for cases of brain radionecrosis. These data were used to derive the neuret formula. Most cases of radiation necrosis occurred for dose/fraction combinations above the line with a slope of 0.44. From Sheline GE, Wara WM, Smith V. Therapeutic Irradiation and Brain Injury. Int J Radiat Oncol Biol Phys. 1980;6(9):1215–1228; used with permission

The influence of fraction size was also assessed by Lee et al. [22] using a product of total dose (D) and fraction size (d) in Gy2. The product (Dd) was found to be the most significant predictive factor for necrosis in a multivariate analysis of seven treatment parameters [22, 23]. A study by Ruben et al. [24] confirms the significance of Dd and also demonstrates that average fraction size ≥2.25 Gy is a significant independent risk factor. Shorter overall treatment time from giving twice daily radiation treatment was also found to be a significant risk factor in the study by Lee et al. [23]
In the 1970s, a linear quadratic (LQ) model was developed to describe cell survival after exposure to ionizing radiation, where the surviving fraction of cells S after exposure to a dose D is represented by
The constant α describes the initial slope of the survival curve at low doses and β describes the quadratic component of cell killing [25]. A common related formula first introduced in the early 1980s[26] is for biologically effective dose (BED):
where D is the total dose and d is the size of a single radiation fraction in Gy. The ratio of α/β has no units, because both α and β are expressed in units of dose in Gy and the ratio tends to be in the range of 2–3 for late effects such as lung fibrosis or CNS injury versus around 8–10 for acute-responding tissues such as hair follicles, mucosa, and rapidly dividing tumor cells, although a considerable range exists. As an example, 50 Gy total dose prescription given at 2 Gy per daily fraction will have a BED value of 100, assuming α/β = 2 (a ratio often used for late effects). A biologically equivalent dose for late effects given at 3 Gy/fraction can be determined by solving the formula 100 = D(1 + 3/2), which gives D = 100/(1 + 3/2) = 40. Thus, 39 Gy at 3 Gy/fraction is approximately equivalent to 50 Gy at 2 Gy/fraction in terms of late effects. As fraction size increases, comparable values for BED for either tumor or late effects can be computed. However, data surrounding the use of this approach with very few fractions or very large dose per fraction are sparse and unreliable.
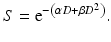
![$$ \mathrm{BED}=D\left[1+\frac{d}{\alpha /\beta}\right], $$](https://radiologykey.com/wp-content/uploads/2017/06/A83315_2_En_65_Chapter_Equc.gif)
Useful tolerance parameters are the 5 and 50 % probabilities of brain injury at 5 years (TD5/5 and TD50/5, respectively). At “standard” fractionation of 1.8–2.0 Gy per fraction, the TD5/5 is estimated to be 50 ± 10 Gy for the whole brain, 60 ± 10 Gy for a portion of the brain, and 45–50 Gy for a 10-cm segment of spinal cord [27, 28]. Thresholds of 54 [29] and 57.6 Gy[30] have been reported for cerebral radiation necrosis, but it is apparent that radionecrosis may occur below these limits with conventional therapeutic radiation [5, 31]. In the North Central Cancer Treatment Group low-grade glioma trial, patients who received 64.8 Gy with conventionally fractionated radiation had a significantly higher actuarial incidence of necrosis (6 patients out of 102) than those who received 50.4 Gy, and only one patient (out of 101) treated with 50.4 Gy in 28 fractions developed radionecrosis [31]. In a report by Marks et al. [29], only 1 patient out of 139 developed necrosis in an area irradiated to <50 Gy in 27 fractions. In the study by Sheline et al. [5], only 3 of 80 cases of brain radionecrosis were in patients receiving doses ≤50 Gy, in fraction sizes <2.5 Gy. In contrast, 17/20 cases of radionecrosis occurring at ≤50 Gy were associated with fraction sizes ≥2.5 Gy. This implies the relative safety of doses in 50 Gy range, but only so long as fraction sizes are maintained under 2.5 Gy.
In single-fraction radiosurgery, the risk of brain necrosis increases rapidly above 12 or 13 Gy, and there is a higher risk with larger nontarget volume encompassed by the 12-Gy isodose line, particularly over 10 mL [32, 33]. Although the LQ model and BED concept are presumed to “break down” in the context of single fraction radiosurgery, it is noteworthy that a single fraction of 13 Gy would yield a BED of 97.5, assuming an α/β ratio of 2, very similar to the 100 Gy BED value obtained with 50 Gy in 2 Gy fractions, with both representing lower, relatively “safe” thresholds. A dose-finding protocol, Radiation Therapy Oncology Group 90–05, found a similar relationship between higher risk of CNS toxicity from single-fraction radiosurgery and larger tumor volume, with lower “maximum tolerated doses” for larger tumors [34].
Latency
The mean interval from the end of radiotherapy until onset of necrosis was 11.6 months in a recent study [24], though radiation necrosis has been reported to manifest as early as 3 months [35] and as late as 47 years after radiotherapy [36]. Peritumoral parenchyma seems to be more vulnerable to damage from radiation, and the potential role of chemotherapy in enhancing this effect was suggested by the observation that the latency period for the development of radionecrosis in glioma patients (typically treated with chemoradiation) appears to be approximately five times shorter than in other patients receiving equivalent radiation dose, such as those with nasopharyngeal carcinoma, often treated with radiotherapy alone [5, 23, 31, 37, 38]. An alternative explanation for this comes from the observation that higher rates of radiation necrosis from radiosurgery are observed when treating recurrent malignant gliomas as opposed to brain metastases, and the putative hypothesis is that malignant gliomas are endowed with a significantly more disrupted and leaky peritumoral vasculature, with endothelium that is more susceptible to damage from large-fraction radiation. Indirect support for this stems from studies of aggressively hypofractionated chemoradiotherapy for glioblastoma in which the concomitant use of bevacizumab, known to stabilize the endothelium, significantly reduced observed rates of necrosis (relative to historic expectations) [39].
Incidence
The incidence of cerebral radiation necrosis after conventional radiotherapy for primary brain tumors in modern radiation oncology practice is poorly defined. This is partially due to limited data on radiation dose-fractionation schedules and lack of both the numerator and the denominator for patient populations within which cases of radiation necrosis are described [5]. Additional barriers relate to the difficulty of distinguishing necrosis from tumor recurrence by neuroimaging and the low rates of reoperation and autopsy in these patients [35] and to limited survival time in patients with malignant brain tumors. Despite advances in magnetic resonance imaging (MRI) and functional imaging, necrosis or necrosis combined with tumor is still probably frequently mistaken for tumor progression alone, likely resulting in underestimation of the true incidence of brain radionecrosis.
A recent review of 426 patients treated for glioma and followed until death or for at least 3 years identified 21 patients with cerebral radionecrosis [24]. Most patients were treated with conventional radiotherapy along with chemotherapy, but ten of the patients who developed radionecrosis had additional boost or salvage stereotactic radiation, usually 18 Gy in three fractions. The crude radionecrosis incidence was 4.9 % and the actuarial incidence was 2.9, 5.1, 9.3, and 13.3 % at 6, 12, 24, and 36 months post-treatment, respectively [24]. When the analysis was restricted to patients treated with a radiation dose biologically equivalent to at least 45 Gy in 25 fractions, the crude incidence of radionecrosis increased to 6 %.
The use of radiosurgery or interstitial radiation after conventional radiotherapy clearly increases the risk of radionecrosis, though reporting rigor, fidelity of follow-up, technique variability and patient population heterogeneity complicate estimation of the rate of radionecrosis, which ranges from 2.6 to 56 % in reported series [29, 31–34, 40–44].
It is important to note that many studies of brain radionecrosis include patients treated with chemotherapy in addition to radiotherapy. Although some investigators have suggested that injury is more often related to radiation than chemotherapy [45], others reported changes similar to “radiation” necrosis from treatment with chemotherapy only for extracranial malignancies [46]. It is now widely accepted that both treatment modalities are injurious to normal brain, with the risk of necrosis increasing when they are used together.
Histopathologic Features
The end point of irreversible radiation injury in the brain is necrosis that may develop months to years after the completion of radiation therapy [3, 16]. Histopathologically, radiation necrosis occurs most commonly in the white matter around blood vessels. The most frequent histopathologic feature is fibrinoid necrosis of blood vessel walls with surrounding perivascular parenchymal coagulative necrosis with or without an inflammatory response (sometimes referred to as “dirty necrosis”) [47]. Focal radiation necrosis may be peritumoral or distant, as well as unifocal or multifocal [12]. Extension and confluence of multiple perivascular foci of necrosis result in large serpentine or confluent zones of parenchymal necrosis [3, 48]. Over time, dystrophic calcifications can develop in these necrotic areas. An additional vascular lesion that is often observed consists of clusters of abnormally dilated, thin-walled telangiectasias. Late vascular changes include vessel wall thickening caused by hyalinization, with resultant luminal narrowing, and ultimately, in some cases, aneurysmal dilation. White matter changes may also be focal or diffuse; in the diffuse pattern, widespread periventricular demyelination is seen [16].
Diagnosis
Radiation necrosis should be in the differential diagnosis for any patient with progressive neurological symptoms or a new or enlarging enhancing brain lesion with a history of prior intracranial radiation therapy to a dose of at least 12 Gy in a single dose (Fig. 65.2) or 50 Gy with at 1.8–2.0 Gy per daily fraction. A major dilemma in the diagnosis of radiation necrosis is differentiating it from recurrent or persistent tumor. Tumor recurrence and radiation necrosis often have a similar appearance on conventional contrast-enhanced MRI [49, 50]. Because of this, it is often difficult to distinguish which entity is responsible for the appearance of an enlarging lesion. Traditionally, a patient’s clinical course, biopsy, and serial imaging for several months have been used to try to distinguish between tumor recurrence and radionecrosis [49–51]. Any investigation short of extensive tissue examination lacks diagnostic sensitivity and specificity. Anatomic and physiologic MRI, positron emission tomography (PET), and thallium single photon emission computed tomography (SPECT) imaging have all been used in an attempt to define the best noninvasive method to diagnose radiation necrosis, though there is no evidence to date that any of these investigations is clearly superior to the other modalities.
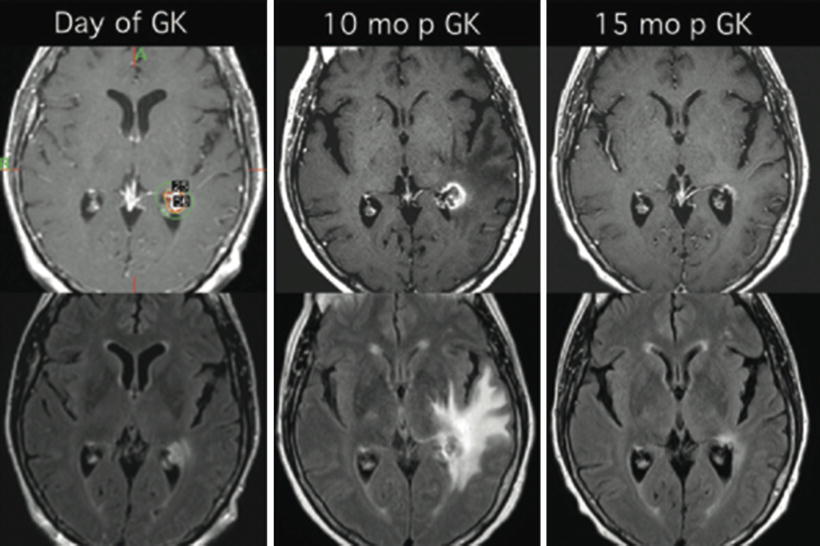
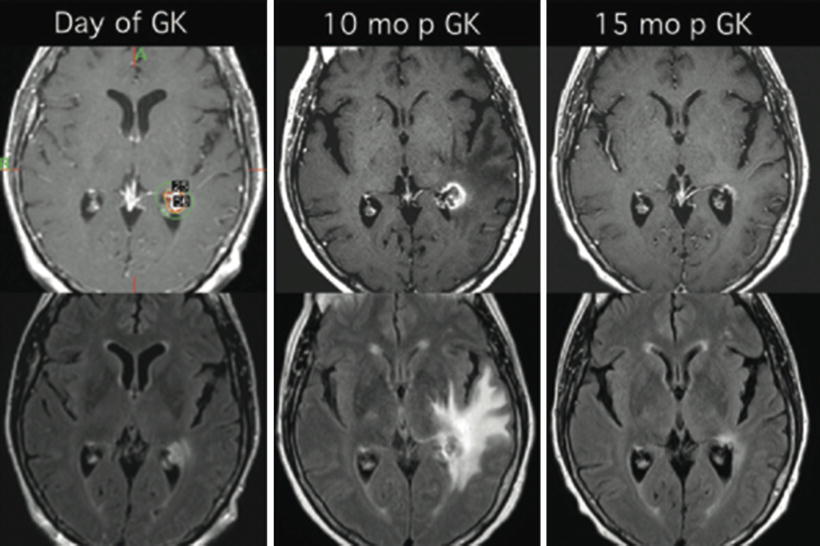
Fig. 65.2
T1-weighted, post-contrast MRI (upper panels) and FLAIR images (lower panels) for a patient with a breast cancer brain metastasis treated with Gamma Knife radiosurgery with a single fraction of 18 Gy, prescribed to 50 % isodose line (upper, left panel). Symptomatic radiation necrosis developed 10 months later (center panels). Five months after the onset of necrosis, the patient underwent a 2-month long treatment with dexamethasone resulting in significant clinical and radiographic improvement (right panels). Symptoms resolved rapidly after initiation of dexamethasone therapy and did not recur after discontinuation of steroids
MRI
Radiation necrosis can closely resemble recurrent tumor on anatomic (“conventional”) MRI because of the following shared features: (a) focus at or close to the original tumor site, which is commonly the site of maximum radiation dose, (b) contrast enhancement, (c) progressive enlargement, at least over several months, (d) surrounding edema, and (e) exertion of mass effect [52]. On MRI, brain necrosis usually consists of a somewhat irregular rim-enhancing mass with a central area of low signal on T1-weighted images (Fig. 65.2, upper center panel). The contrast enhancement of these lesions is thought to be secondary to radiation-induced endothelial damage resulting in breakdown of the blood–brain barrier. On T2-weighted images, the surrounding edema has high signal intensity, the solid portion of the radionecrotic lesion has low signal intensity, and the central necrotic component may show either increased or decreased signal intensity (Fig. 65.2, lower center panel). Later, focal brain atrophy develops, and sometimes large cysts develop. The arcuate fibers in white matter are relatively resistant to radiation necrosis and are usually involved late in the disease process. The predilection for periventricular white matter involvement in radiation necrosis may, on some occasions, mimic multiple sclerosis. Multiple lesions can resemble multiple metastases, and radiation-induced necrotic lesions can also spread subependymally and mimic subependymal tumor spread.
Despite the many commonalities in radiographic appearance between tumor and necrotic tissue, several investigators have attempted to define patterns of radiation necrosis on conventional MRI with the aim of distinguishing tumor from necrosis. A recent study of 59 patients treated with radiosurgery for metastatic brain tumors who underwent subsequent craniotomy for symptomatic lesion enlargement analyzed the following features: (a) arteriovenous shunting, (b) gyriform lesion or edema distribution, (c) perilesional edema, (d) cyst formation, and (e) pattern of enhancement [53]. The authors also defined a radiographic feature, the lesion quotient, which is the ratio of the nodule as seen on T2 imaging to the total enhancing area on T1 imaging, and suggested that a lesion quotient ≥0.6 correlates with recurrent tumor, whereas a quotient of ≤0.3 correlates with radionecrosis. The examined radiographic features, taken together, achieved 80 % or greater predictive value but had either low sensitivity or low specificity [53]. In an older study, Kumar et al. [12] cite two radiographic features that are consistent with radiation necrosis, the “Swiss cheese” pattern and the “soap bubble” pattern. The first pattern is characterized by diffuse areas of enhancement affecting the cortex and white matter and intermixed with necrotic areas. The soap bubble appearance refers to smaller lesions with heterogeneously enhancing necrotic cores.
The key point is that radiation necrosis should be included high in the differential diagnosis if some of the described imaging features or patterns are recognized. However, no radiographic pattern is pathognomonic for radiation necrosis and as such, supplementary functional imaging is often necessary and even then, surgical excision may still be required.
MRS
Magnetic resonance spectroscopy (MRS) has been explored as a noninvasive means of diagnosing radiation necrosis and distinguishing it from tumor recurrence. The technique allows metabolites from an area of interest to be quantified and compared with those of surrounding normal tissue [54]. Metabolites such as N-acetyl aspartate (NAA), phosphorylcholine/glycerophosphorylcholine (Cho), creatine (Cr), lactate (Lac), and mobile lipids (Lip) provide different spectra in tumors compared with normal brain or radiation necrosis. Elevated levels of choline compounds have been repeatedly reported in the “peritumoral region” for gliomas [55–59] but not metastases [56].
In general, tumor is characterized by NAA/Cho ratio <1 and Lip/Cho ratio <3. In contrast, Lip/Cho ratio >3 or significant decrease of all metabolite levels within the lesion or surrounding brain are considered characteristic of radiation-induced necrosis [60]. The technique has evolved significantly from single-voxel, low-resolution studies to multi-voxel acquisitions that have a much higher resolution [60, 61].
DWI
Diffusion-weighted imaging (DWI) is another interesting approach for utilizing MR technology to diagnose radiation necrosis. The technique depends on the diffusion of water molecules within the tissue under study and generates an apparent diffusion coefficient (ADC) map [54]. Areas with high ADC appear dark on DWI whereas low ADC areas produce increased signal. The differences in ADC in various tissues are thought to result from both changes in the balance between intracellular and extracellular water and changes in the structure of the two compartments. In theory, areas of radiation necrosis should have higher (or better) diffusion coefficients than areas of hypercellular tumor so that the technique could be used to differentiate the two conditions. This explanation represents a gross simplification of the biologic milieu of both tumor and necrosis, but high diffusion on ADC has been reported in cerebral radiation necrosis caused by treatment of brain tumors [66–69].
Previous studies applied this technique to evaluate cellularity in gliomas with the aim of predicting tumor grade [70]. Signal intensity and ADCs in neoplastic tissue, peritumoral edema, and normal brain tissue have been reported for low- and high-grade gliomas, meningiomas, and brain metastases [71]. Necrotic brain tissue in the temporal lobe after radiation therapy for nasopharyngeal carcinoma has been characterized using DWI as well [72]. Previously reported ADC values for high-grade glial tumors vary from 1.1 to 1.37 × 10−3 mm/s2 [73–75]. ADCs in peritumoral edema or temporal lobe necrosis were reported as 1.29 and 2.88 × 10−3 mm/s2 in two notable studies [72, 76]. Although ADC maps can provide useful information that may help distinguish tumor from necrosis, DWI has not been studied sufficiently to permit unequivocal diagnosis. Presently, DWI is primarily used as an adjunct to other studies.
Perfusion MRI
Dynamic, contrast-enhanced perfusion MRI (pMRI) of the brain provides hemodynamic information that complements the anatomic information available from conventional MRI. Contrast-enhanced pMRI methods exploit signal changes that accompany the passage of a paramagnetic contrast agent (gadolinium) through the cerebrovascular system to derive relative blood volume and flow information [77, 78]. Analysis of dynamic data from pMRI yields quantitative estimates of the relative cerebral blood volume (rCBV) of the lesion versus other regions of interest such as surrounding tissue or contralateral normal tissue. For CBV measurements, a series of images is acquired at intervals of approximately 1 s before, during, and after bolus injection of the contrast agent. Rapid gradient-echo or echo-planar imaging is generally used to acquire multiple T1-weighted slices at each time point during the imaging interval. When a paramagnetic contrast agent passes through the cerebral vascular system, it induces differences in local magnetic susceptibility between the intravascular space and the surrounding normal tissue, with a transient and differential loss of relaxation signal. This enables calculation of the contrast concentration–time curve, and rCBV can then be estimated from the area under this curve and corrected for contrast leakage using a reference measurement in the contralateral, unaffected centrum semiovale white matter.
Sugahara et al. [79] were among the first to propose that this technique should be utilized when it is necessary to differentiate tumor recurrence from radiation necrosis. In a series of 20 cases, these authors found that a normalized rCBV of 2.6 or higher indicated tumor recurrence and a ratio of 0.6 or less was suggestive of nonneoplastic contrast-enhancing tissue.
A more recent approach, described by Barajas et al. [80], uses three hemodynamic variables to describe the shape of the signal intensity–time curve obtained from dynamic susceptibility contrast pMRI: rCBV, relative peak height, and percentage of signal intensity recovery; among 27 patients with necrosis or tumor recurrence or progression after single-fraction radiosurgery for brain metastases, rCBV and relative peak height were significantly higher and the percentage of signal intensity recovery was significantly lower for tumor versus necrosis [80, 81]. Relative CBV is the most widely used hemodynamic variable derived from pMRI and has been shown to correlate with tumor grade and tumor microvascular attenuation [56, 79, 82–88]. Relative peak height, which represents the maximal change in signal intensity during the passage of contrast agent, has been shown to correlate with rCBV measurements and tumor capillary blood volume. Finally, percentage of signal intensity recovery, an indicator of the blood–brain barrier integrity, reflects the degree of contrast agent leakage through tumor microvasculature and provides insight into the alteration of capillary permeability.
Several important limitations are associated with dynamic, contrast-enhanced pMRI. Because the technique is susceptibility weighted, it is extremely sensitive to structures or lesions that induce strong magnetic field inhomogeneities such as blood products, calcium, melanin, metals, or lesions near brain–bone–air interfaces such as the skull base. When there is a large amount of susceptibility artifact, pMRI fails to provide any meaningful information [89]. Also, the calculation of rCBV can be grossly inaccurate in lesions with a severe breakdown or absence of the blood–brain barrier.
FDG-PET
Positron emission tomography (PET) can be used to measure the metabolism of glucose labeled with an 18 F analog. Because radiation necrosis should be characterized by low glucose consumption, 18 F-fluorodeoxyglucose (FDG) is often used in PET studies to provide contrast with areas of tumor, which should have higher uptake. Early studies reported that 18 F-FDG-PET had a sensitivity of 81–86 % and a specificity of 40–94 % for distinguishing between radiation necrosis and tumor [90].
There are multiple limitations to the usefulness of PET in the brain. Tumor glucose metabolism is highly variable, and the overlap of 18 F-FDG uptake between radiation necrosis and tumor can be considerable [91–93]. In addition, tumor glucose metabolism is frequently lower than that of normal brain tissue; thus, tumor uptake may be difficult to appreciate amid the background of highly metabolically active normal brain tissue or of treated brain tissue, which tends to have lower glucose metabolism than untreated brain and may also have a wide range of metabolic activity. Also, high 18 F-FDG uptake can occur from inflammation that may be associated with necrosis, resulting in misinterpretation. In general, it has not been possible to define a reliable cutoff of standardized uptake values to distinguish between tumor and necrosis. Strategies to improve predictive value include comparing the ratio of the lesion to contralateral normal white or gray matter or determining whether lesion activity is above the expected background activity in adjacent brain tissue [94–97]; in either case, it can be helpful to have the anatomic information from coregistration with MRI [97, 98].
Amino Acid PET
Amino acid PET tracers and amino acid analog PET tracers constitute another class of tumor imaging agents [99, 100]. These are particularly attractive for imaging brain tumors because of the high uptake in tumor and low uptake in normal brain tissue; amino acids are transported into the cell via carrier-mediated processes [100], and amino acid transport is generally increased in malignant cells [101, 102]. The most extensively studied amino acid tracer is 11C-methionine [103]. Because of the short half-life of 11C (approximately 20 min), 18 F-labeled aromatic amino acid analogs (with a half-life of approximately 109 min) have been developed for tumor imaging [104].
Because amino acid tracers appear more sensitive than 18 F-FDG-PET in visualizing tumor, they also have potentially better diagnostic accuracy in evaluating radiation necrosis. However, the degree of amino acid uptake in radiation necrosis is not known. 11C-methionine has been available for about two decades, but few studies have been published on its performance in differentiating radiation necrosis from brain tumor recurrence.147 In a study of 21 patients with brain metastases treated with SRS, 11C-methionine correctly identified seven of nine recurrences and 10 of 12 radiation injury lesions [95].
In rats, uptake of 18F-FET (18 F-fluoroethyl-l-tyrosine), 18 F-FDG, and 18 F-choline has been compared in acute lesions caused by cerebral irradiation and cryotherapy [105]. Both 18 F-FDG and 18 F-choline accumulated in macrophages, a common inflammatory cell type in radiation necrosis, but 18 F-FET uptake was absent from macrophages. Moreover, the ratio of 18 F-FET uptake in radiation necrosis to that in normal cortex was much lower than the corresponding ratios for 18 F-FDG and 18 F-choline, suggesting that 18 F-FET may be able to differentiate radiation necrosis from tumor recurrence. Absence of 18 F-FET uptake in a case of radiation necrosis has been reported [106]. Although these results are promising, larger systematic studies are needed to evaluate the diagnostic performance of these tracers.
SPECT
SPECT relies on the injection of a radionuclide such as 201Tl (Thallium-201) and 99mTc (Technetium-99) and the use of a gamma camera and computed tomography (CT) to detect its distribution in the brain. Uptake of the radionuclide is greater in tumor cells than in normal tissue and inflammatory cells and depends on Na/K-ATPase pump activity [107]. Because uptake requires a viable cell, it is correspondingly low in necrotic areas. This technique is much less expensive and more widely available than PET but has disadvantages with regard to limited breadth of molecular processes that are accessible, quantitation accuracy, and spatial resolution.
High-grade tumors have a higher thallium index than low-grade tumors. In a series of 15 biopsy-proven cases, Schwartz et al. [108] found excellent correlation between imaging and histology (14/15 cases) using dual-isotope SPECT scanning with 201Tl and 99mTc-hexamethylpropylene amine oxime (HMPAO).161 A subsequent study revealed high 201Tl uptake in recurrent tumor and low uptake in radiation necrosis. In cases with intermediate uptake, 99mTc-HMPAO uptake helped to differentiate tumor recurrences from radiation necrosis by showing increased perfusion in tumor [108]. After these initial exciting reports of sensitivities in excess of 90 % for detecting tumor recurrence with specificity approximately 60 % [109], other reports citing concerns about the diagnostic accuracy of the technique for distinguishing recurrent tumor from radiation necrosis began to surface, including cases of thallium-avid radiation necrosis [110–112].
More recently, Alexiou et al. [113] substantiated potential superiority of 99mTc-tetrofosmin (99mTc-TF) over 99mTc-sestamibi, a commonly used imaging agent, for brain tumor imaging, owing to the fact that 99mTc-TF accumulation is independent of the multidrug-resistance phenotype of some glioma cells (167,168).99mTc-TF is a tumor-seeking diphosphine that does not cross the intact blood–brain barrier, and uptake is dependent on regional blood flow and cell membrane integrity, thus reflecting cellular metabolic status and viability. Alexiou et al. also reported that 99mTc-TF could successfully differentiate tumor recurrence from radiation injury [113, 114]. These results are yet to be confirmed by other groups.
Histological Examination
Given the limitations of the various imaging techniques, a definitive diagnosis of radiation necrosis may require pathologic examination of surgical specimens. Because of the potential for sampling error with biopsy alone, total resection is preferred. Resection also improves edema and mass effect associated with radiation necrosis and/or active tumor. If resection is not safe or feasible, the imaging modalities described above may be used to guide biopsies, so that the region most suspicious for active tumor can be sampled to maximize diagnostic yield.
Treatment
While the finding of radiation necrosis, or “treatment effect,” is often met with relief that the changes seen on imaging do not represent tumor growth, patients and their families and health care providers need to understand the implication of this condition. Radiation necrosis can produce symptoms that are as disabling as tumor recurrence. Most patients with radiation necrosis present with focal neurologic deficits related to the necrotic lesion as well as headaches as a result of mass effect from reactive vasogenic edema. Some cases stabilize or improve with the treatments described below or with the passage of time, but some cases may be relentlessly progressive.
Steroids. Steroids are frequently used to treat radiation-induced edema and necrosis. Animal models of radiation-induced CNS necrosis demonstrate that edema precedes the development of necrosis and that the anti-inflammatory effect of dexamethasone, when provided early, can modify subsequent vascular and inflammatory changes to reduce and delay the development of subsequent necrosis [115–117]. Thus, controlling edema after irradiation or in the early stages of necrosis may limit the subsequent development or course of necrosis. Among patients with temporal lobe necrosis after radiotherapy for nasopharyngeal cancer, dexamethasone given at the onset of radiation necrosis, during which there was marked reactive edema, resulted in significant objective improvement of necrosis in 38 % of patients, whereas none of the patients with cystic necrosis improved [118].
The optimal dose and timing of the corticosteroids have not been prospectively studied; however, it appears that administration early in the course of radionecrosis is more effective than one that is delayed, and the doses given must be adequate to control clinical symptoms. After symptoms improve, the steroid dose should be tapered as tolerated. Some patients become symptomatic when steroid treatment is discontinued and thus require long-term treatment over many weeks or months. Attention must then be directed toward minimizing the medical complications associated with steroid treatment, such as steroid myopathy, glucose intolerance, secondary infections, gastric irritation, neuropsychologic issues, and osteopenia.
Surgical Resection. Radiation-induced necrosis is clearly a dynamic process. Cases have been documented in which necrosis spontaneously resolves, remains stable, or progressively enlarges to produce a space-occupying lesion that becomes increasingly symptomatic. When steroid therapy is poorly tolerated or when radiation necrosis becomes progressively larger and symptomatic despite the use of steroids, surgical excision of the necrotic mass is indicated [3, 117, 119]. In general, surgical treatment is an effective therapy for well-circumscribed areas of necrosis and much less effective for diffusely necrotic lesions [120]. It is presumed that removal of the necrotic nidus, a source of inflammation, reduces the associated edema and thereby secondary mass effect. Surgery thus provides immediate relief of symptoms and frequently enables patients to be rapidly tapered off steroids.
Hyperbaric Oxygen (HBO). HBO therapy is the inhalation of 100 % oxygen at an elevated pressure, usually over 1.5 times the atmospheric pressure, in a controlled and monitored environment. When used after radiation therapy, HBO has been shown to increase tissue oxygenation and angiogenesis and improve capillary bed function [121]. HBO has been used in the treatment and prevention of late complications after radiotherapy in different sites, and many papers have been published reporting its use [119]. Hulshof et al. [122] prospectively evaluated HBO in patients with cognitive problems following cerebral radiotherapy and found that only one out of seven patients experienced significant improvement. Leber et al. [123] reported two cases of successful HBO therapy for radiation damage following Gamma Knife radiosurgery for AVMs, with marked improvement in necrotic lesions on imaging without the use of corticosteroids. Given the scarcity of evidence and lack of controlled trials, there is considerable uncertainty on the place of HBO in the management of late neurological sequelae of radiation therapy.
Anticoagulants. Because it is thought that radiation necrosis results mainly from vascular changes associated with ischemia, anticoagulants (heparin and warfarin [Coumadin]) and antiplatelet medications such as pentoxifylline (Trental), aspirin, and ticlopidine (Ticlid) have been used to attempt to halt its progression. Five of eight patients with cerebral radiation necrosis unresponsive to steroids had clinical improvement on anticoagulation with heparin (Heparin) and warfarin (Coumadin) [124]. The potential risk of bleeding from these agents must be weighed against any expected benefit, and these patients must be closely monitored for consumptive coagulopathies, especially in the setting of concurrent chemotherapy. Definitive conclusions are not available owing to the small number of patients studied. However, in cases where radiation necrosis is progressive and symptomatic, full anticoagulation is reasonable assuming there are no contraindications. There are no published comparisons for antiplatelet versus anticoagulant agents.
Antioxidant Therapy. Oral pentoxifylline (Trental) and tocopherol (vitamin E) combination therapy has been reported to benefit patients with skin and chest wall radionecrosis as well as mandibular osteoradionecrosis [125]. Pentoxifylline is thought to reverse radiation damage primarily through its ability to increase locoregional blood flow; it improves red blood cell deformability and promotes laminar blood flow by inhibiting intercellular adhesion molecule expression, resulting in decreased adhesion between endothelial cells and leukocytes. Pentoxifylline also decreases plasma fibrinogen while increasing fibrinolytic activity [126], and it is a nonspecific inhibitor of many inflammatory cytokines [127]. Tocopherol is an antioxidant compound that scavenges ROS generated during oxidative stress and protects lipid membranes against lipid peroxidation [125]. Williamson et al. [128] reported on 11 patients with delayed adverse radiation effects after treatment with Gamma Knife radiosurgery for various benign and malignant disorders who were treated with pentoxifylline (400 mg orally twice daily) and tocopherol (400 IU orally twice daily). The edema volume measured on serial FLAIR-MRI improved in 10 of 11 patients, with an average volume decrease of 72.3 mL. The only patient without improvement was found to have tumor recurrence. The authors concluded that pentoxifylline and tocopherol may be of benefit for treatment of adverse radiation effects. Further studies are needed to determine the efficacy of this regimen for the treatment of brain radionecrosis.
Bevacizumab. The premise for the use of bevacizumab for radiation necrosis came from observations of radionecrosis-associated endothelial cell dysfunction and hypoxia with liberation of vasoactive compounds, including vascular endothelial growth factor (VEGF). Bevacizumab is a humanized murine monoclonal antibody against the VEGF molecule. It blocks VEGF from reaching its capillary targets and is currently being used in the treatment of several solid tumors. Gonzalez et al. [129] retrospectively evaluated 15 patients treated with bevacizumab or bevacizumab combined with chemotherapy after radiation for malignant brain tumors. Radiation necrosis was diagnosed in eight patients, all of whom underwent therapy with bevacizumab on either a 5 mg/kg/2-weeks or 7.5 mg/kg/3-weeks schedule. Posttreatment MRI scans, performed approximately 8 weeks after therapy, demonstrated measurable improvements in all patients. The imaging findings correlated with clinical improvement as evaluated by the average reduction in daily dexamethasone requirement by 8.6 mg. The authors of this study concluded that bevacizumab, alone or in combination with other agents, can reduce radiation necrosis by decreasing capillary leakage and the associated brain edema. Torcuator et al. [130] reported on six glioma patients with biopsy-proven brain radionecrosis treated with bevacizumab at 10 mg/kg every other week, combined with irinotecan in two cases of suspected tumor cells in addition to necrosis. Compared with pre-bevacizumab imaging, the mean decrease in volume of contrast enhancement was 79 % and the mean decrease in T2-FLAIR volume was 49 %. Similar results were published in a recent case report of bevacizumab at 5 mg/kg every other week for temporal lobe necrosis that developed 2 years after aggressive radiotherapy with chemotherapy for nasopharyngeal carcinoma [131] and in three of four children with pontine gliomas suspected of having radiation necrosis [132]. More recently, Gutin et al. [39] reported no cases of radionecrosis among 25 patients with recurrent malignant gliomas treated with fractionated stereotactic reirradiation to 30 Gy in six fractions along with bevacizumab, 10 mg/kg every 2 weeks. Optimal dosing, duration of treatment, and efficacy of bevacizumab (or similar agents) need further evaluation in prospective trials.
Rehabilitation. Depending on the symptoms and clinical deficits caused by radiation necrosis, patients may benefit from physical, occupational, or speech therapy.
Brain necrosis is associated with a significant degree of morbidity and sometimes mortality. It has been recognized as a potential complication of brain radiation therapy for at least five decades, with higher risk for larger volumes, higher total dose, and higher dose per fraction, especially above about 50 Gy at 1.8–2.0 Gy per daily fraction or 12 Gy in one fraction (three- and five-fraction radionecrosis thresholds are not well know at this time) (see Table 65.1). The latency period is highly variable, but the minimum latency is about 3 months after radiation exposure. The pathophysiology is not completely understood; contributing factors appear to include injury to vasculature, oligodendrocytes, and/or neural stem cells and chronic inflammation. It may be difficult to distinguish radiation necrosis from progressive or recurrent tumor following treatment for primary or metastatic brain tumors. Techniques that have been used include MRI, MRS, PET, and SPECT, short of surgical biopsy or resection for a histopathologic diagnosis. The symptoms of radiation necrosis may be mild or reversible, but are sometimes significant or progressive, warranting treatment with steroids and/or surgical resection. Less well-proven but possibly effective interventions include HBO therapy, anticoagulation, antioxidant therapy, and therapy with VEGF inhibitors.
Table 65.1
Summary of suggested dose constraints for selected critical brain structures
Tissue | Max critical volume above threshold | One fraction | Three fractions | Five fractions | End point (>-Grade 3) | |||
---|---|---|---|---|---|---|---|---|
Threshold dose (Gy) | Max. point dose (Gy) | Threshold dose (Gy) | Max. point dose (Gy) | Threshold dose (Gy) | Max point dose (Gy) | |||
Optic pathway | <0.2 cm3 | 8 | 10 | 15.3 (5.1 Gy/fx) | 17.4 (5.8 Gy/fx) | 23 (4.6 Gy/fx) | 25 (5 Gy/fx) | Neuritis |
Cochlea | 9 | 17.1 (5.7 Gy/fx) | 25 (5 Gy/fx) | Hearing loss | ||||
Brainstem (not medulla) | <0.5 cm3 | 10 | 15 | 18 (6 Gy/fx) | 23.1 (7.7 Gy/fx) | 23 (4.6 Gy/fx) | 31 (6.2 Gy/fx) | Cranial neuropathy |
Spinal cord and medulla | <0.35 cm3 | 10 | 14 | 18 (6 Gy/fx) | 21.9 (7.3 Gy/fx) | 23 (4.6 Gy/fx) | 30 (6 Gy/fx) | Myelitis |
<1.2 cm3 | 7 | 12.3 (4.1 Gy/fx) | 14.5 (2.9 Gy/fx) | |||||
Spinal cord subvolume (5–6 mm above and below level treated per Ryu) | <10 % of subvolume | 10 | 14 | 18 (6 Gy/fx) | 21.9 (7.3 Gy/fx) | 23 (4.6 Gy/fx) | 30 (6 Gy/fx) | Myelitis |
Complications for Common Radiosurgery Indications
Brain Metastases. Metastases to the brain represent the most common indication for radiosurgery [6]. In a series evaluating radiosurgery for brain metastases, Shaw et al. report that the most common complication was symptomatic cerebral edema associated with radiosurgery [8]. Four patients in this series experienced severe cerebral edema, two of whom responded to corticosteroids. Six percent of patients in this series required surgical resection for persistent and symptomatic radionecrosis within 1 year of SRS. These investigators noted that larger target volume >8.2 cm3 was significantly associated with increased acute and delayed complications due to radiosurgery [8]. In a different series of 40 patients with intracranial metastasis, Mehta et al. [133] reported a 10 % radiation toxicity rate associated with radiosurgery.
Since most patients with brain metastases are exposed to various systemic therapies, often involving novel targeted agents, radiosensitizing effects of these new agents are not well known and understood. There are some case reports suggesting enhanced cutaneous toxicity with the combination of BRAF inhibitors and radiotherapy but whether these effects translate into greater neurotoxicity is not known [134].
Symptomatic cerebral edema is the most commonly reported complication of SRS for intracranial metastases with a range of 3–18 % [8, 135]. Typically, these complications occur over a broad time period. Focal symptoms are usually closely related to the location of the injury and/or to increased intracranial pressure. Whenever a patient becomes symptomatic after radiosurgery with a headache, seizure, or progressive neurologic deficit, the interval since radiosurgery and the clinical setting during which radiosurgery was performed must first be considered. For example, was the patient treatment with radiosurgery alone or after whole-brain radiotherapy (WBRT)? Apart from radiation side effects, tumor recurrence and growth must also be considered as possible causes of symptoms. Acute side effects in the first few hours or days after radiosurgery can be treated symptomatically for complaints of pain, nausea, and fatigue. Seizures are quite rare in the acute setting, but if they occur, anticonvulsant levels should be checked (if applicable), dose adjusted (an additional half-loading dose given [orally or IV] and the maintenance dose increased pending the results), or the patients should be converted to anticonvulsants not metabolized through the hepatic system (e.g., levetiracetam). If the time from radiosurgery is weeks to months, then edema, necrosis, tumor recurrence, and/or hemorrhage as causes of symptoms should be considered. Evaluation in this time period almost always necessitates evaluation with a CT scan (if acute onset and suspicion of cerebral hemorrhage is high; melanoma, renal cell carcinoma, and uterine carcinoma metastases have higher propensity to bleed) or MRI. If symptoms are relatively mild, then outpatient treatment with corticosteroids (or increasing the dose) is a reasonable first step. If there is no improvement in symptoms within several days despite increased dose of steroids, then repeat MR imaging is indicated to help distinguish radionecrosis from tumor or rule out other causes of symptoms. If symptoms resolve with steroids and the medication can be tapered and withdrawn without symptomatic recurrence, then no further intervention is required. Because long-term high-dose steroids can cause a host of problems including hyperglycemia, muscle weakness, Cushingoid facies, insomnia, irritability and fatigue, other alternatives need to be investigated depending on the cause of symptoms (see Fig. 65.3).