Contrast Enhancement: Differentiating Tumor Recurrence from Non-Neoplastic Tissue following Intervention (Surgery, Chemotherapy, Radiotherapy)
Jalal B. Andre
James Fink
Mark S. Shiroishi
Pia C. Sundgren
Sarah Foster
Alexander Lerner
Michael T. Booker
Treatment strategies for newly diagnosed brain tumors vary by tumor histology, tumor grade, and whether the disease arises primarily within the central nervous system (CNS), as with gliomas, or systemically within the body with secondary CNS involvement, as with metastases.1,2 Nevertheless, the goals of therapy are essentially the same: maximize the removal of tumor tissue while preserving the integrity and function of normal CNS structures. The extent to which this can be successfully achieved through surgical intervention depends on whether the location of the tumor is amenable to safe, complete resection and whether the tumor cells invade the surrounding normal parenchyma. In general, with rare exceptions, metastatic tumors within the brain do not invade the adjacent normal tissue, whereas diffusely infiltrative malignant gliomas are known to invade the CNS and extend beyond even the visible abnormalities seen on magnetic resonance imaging (MRI).3,4,5,6 Local control of metastatic disease can, therefore, be achieved by surgical resection and/or stereotactic radiosurgery, as opposed to glioblastoma and other malignant gliomas wherein local recurrences are common even after initial gross total resection. If metastatic lesions are too widespread within the CNS, such that surgery or radiosurgery are impractical (e.g., leptomeningeal carcinomatosis or a large number of CNS metastases from hematogenous spread), then whole-brain radiation or craniospinal radiation is often the mainstay of CNS treatment along with chemotherapy for treatment of systemic disease.
For primary brain tumors, the treatment strategy depends upon the tumor histopathology and grade.1 From a practical standpoint, these histopathologic features are unknown to the surgeon at the initial presentation of a newly diagnosed brain tumor, therefore, the decision to biopsy or resect is informed by the tumor location within the brain and the extent of disease at the time of surgery. Although the importance of achieving gross total resection of malignant gliomas remains controversial, there has been some accumulation of evidence that maximal safe removal leads to improved survival in both high grade7,8,9,10,11 and low-grade12,13,14,15 gliomas. Therefore, the ability to accurately distinguish gross total resection (generally defined as complete removal of abnormal enhancing tumor or complete removal of solid-appearing, nonedema-like T2-weighted signal abnormality if the tumor does not enhance) from near-complete or subtotal resection of malignant gliomas by imaging is relevant to prognosis and treatment planning.16 For the neuroradiologist, making this distinction begins with obtaining postoperative conventional anatomic gadolinium-enhanced MRI and carefully comparing the pre- and postcontrast T1-weighted sequences with the preoperative scans for any residual disease (Fig. 59.1). A surgeon’s own intraoperative assessment of resection completeness without the support of imaging is not sufficient to determine the presence or absence of residual disease or the extent of resection.16,17 Where intraoperative MRI or intraoperative fluorescence using 5-aminolevolinic acid are available, these techniques can be performed at the time of surgery to verify the extent of resection prior to closure, although defined benefits from these practices beyond standard of care resection with intraoperative MRI neuronavigation has yet to be established.18,19,20,21 In cases of neurosurgical resection, regardless of whether a gross total resection has been achieved, the initial postoperative MRI scan establishes a baseline assessment of residual disease for treatment planning, including radiotherapy targeting. The initial postoperative scan is typically obtained within 48 to 72 hours after surgery to reduce the possibility of enhancement due to inflammatory postsurgical change, which can confound the assessment of extent of resection in the case of enhancing tumors.22,23 When stereotactic biopsy is performed for tissue diagnosis without further surgical intervention, as in the case of inoperable tumors, many centers will obtain a postoperative computed tomography (CT) scan to assess for acute hemorrhage; therefore, the preoperative MRI scan obtained for surgical planning and intraoperative guidance most often serves as the baseline for planning of therapy in such biopsy-only cases.
Although involved field radiation therapy combined with temozolomide remains the standard of care for initial glioblastoma treatment,24 there has been a recent increase in the number of clinical trials for patients with high-grade gliomas owing to the development of molecular tests for cancer-related genomic changes and the identification of multiple novel therapeutic targets.25,26 Moreover, the precise treatment goals and timing of radiation therapy for treatment of diffusely infiltrative low-grade glioma
remains unclear.27 Knowledge of the treatment regimen is relevant to the role neuroimaging plays in glioma treatment monitoring, because the phenomena of pseudo-progression during the early-delayed postradiation period and that of pseudo-response from antiangiogenic therapy have complicated serial follow-up assessments and clinical trial endpoints based on standard gadolinium-enhanced MRI (discussed in further detail below).25
remains unclear.27 Knowledge of the treatment regimen is relevant to the role neuroimaging plays in glioma treatment monitoring, because the phenomena of pseudo-progression during the early-delayed postradiation period and that of pseudo-response from antiangiogenic therapy have complicated serial follow-up assessments and clinical trial endpoints based on standard gadolinium-enhanced MRI (discussed in further detail below).25
![]() FIGURE 59.1. Residual glioblastoma on magnetic resonance imaging 24 hours after subtotal resection. A 56-year-old man with residual glioblastoma following initial subtotal resection surgery. Preoperative axial three-dimensional T1 spoiled gradient echo postgadolinium (A) and T2 fast-spin echo (FSE) (B) sequences obtained for intraoperative guidance show a heterogeneously rim-enhancing mass lesion with surrounding edema centered in the left temporal lobe. Postoperative axial T1 spin echo pre- (C) and postcontrast (D) and T2 FSE (E) images obtained 24 hours after surgery show residual enhancement at the posteromedial margin of the resection cavity, consistent with residual enhancing tumor. Corresponding apparent diffusion coefficient map (F) shows no evidence of restricted diffusion to suggest ischemia or infarct, confirming residual tumor enhancement as opposed to subacute infarct enhancement (see also Fig. 59.3). |
Ideally, radiation therapy should be initiated shortly after surgery once the final diagnosis has been established and a plan for care with radiotherapy has been made. The start of radiation therapy may be delayed by the need for postsurgical wound healing or the logistics of treatment decision making, particularly if central review of tumor histology is required for entry into a clinical trial. In the case of fast-growing tumors, there can be a substantial increase in the amount of residual disease between the baseline postoperative MRI scan and the start of radiation therapy (Fig. 59.2), which may be associated with shorter survival.28 However, conventional anatomic gadolinium-enhanced MRI obtained after surgery may show enhancement at or near the neurosurgical resection site because of postsurgical ischemic changes.29 For this reason, comparison with the apparent diffusion coefficient maps obtained prior to surgery and immediately postoperative may be valuable to distinguish residual tumor from devascularized ischemic tissue at the resection margins (Fig. 59.3). In the case of vascular tumors, MR perfusion imaging may also be helpful as a problem-solving tool to distinguish enhancement caused by blood–brain barrier (BBB) injury within uninvolved tissue from residual or recurrent tumor.28
During the period of radiation therapy (typically 6 weeks with delivery of 54 to 60 Gy, depending on tumor histology and World Health Organization [WHO] grade), imaging with MRI is not routinely performed. Expected changes on MRI during the acute phase of radiation injury may include T2 hyperintensity and possibly gadolinium enhancement on T1-weighted scans owing to
microvascular injury, which are thought to be secondary to BBB breakdown and vasogenic edema.30 MR perfusion performed during this period may provide an early prognostic marker to distinguish between early tumor progression and early-delayed postradiation treatment effects that can occur during the period of early postradiation treatment surveillance, but this technique has not been validated.31 In both radiation necrosis and in pseudo-progression, one would observe a decrease in relative cerebral blood volume (rCBV) compared with normal tissue, which would be lower than that of primary and recurrent tumors.
microvascular injury, which are thought to be secondary to BBB breakdown and vasogenic edema.30 MR perfusion performed during this period may provide an early prognostic marker to distinguish between early tumor progression and early-delayed postradiation treatment effects that can occur during the period of early postradiation treatment surveillance, but this technique has not been validated.31 In both radiation necrosis and in pseudo-progression, one would observe a decrease in relative cerebral blood volume (rCBV) compared with normal tissue, which would be lower than that of primary and recurrent tumors.
Posttreatment surveillance with MRI typically begins 4 weeks after completion of involved field radiation therapy, and this conventional anatomic gadolinium-enhanced MR examination establishes a new baseline of residual disease after cytoreductive radiation or chemoradiation therapy. For posttreatment response assessment with MRI, the Response Assessment in Neuro-Oncology (RANO) criteria provide an update to the previous Macdonald criteria for determination of a patient’s best response to chemotherapy, particularly in the context of clinical trials.27,32,33 As in the Macdonald criteria, the RANO criteria response assessment for high-grade glioma is mainly driven by the presence and size of any gadolinium-enhancing tumor on serial MRI scans (Table 59.1), although no predefined window and level settings have been agreed upon, adding the potential of error to the measurement process.
In the case of measurable disease (i.e., lesions with clearly defined margins and a diameter of at least 1 cm), each measurable lesion is typically measured in two dimensions (longest axis and orthogonal short axis) using
the RANO criteria. If there are multiple measurable lesions, then at least two lesions are measured, the products of these dimensions are summed, and the sum total of area-product measurements is taken to represent the burden of residual enhancing tumor. In this context, an increase of gadolinium-enhancing disease on T1-weighted scans by at least 25%, the appearance of any new lesions, the clear progression of nonmeasurable disease, a clear increase in size of T2/fluid-attenuated inversion recovery (FLAIR) signal lesions, or a clear decline in clinical status represents progressive disease. A decrease in gadolinium-enhancing disease on T1-weighted scans by at least 50%, with stable size of T2/FLAIR signal abnormality, no new lesions, stable or decreasing corticosteroid therapy, and stable or improved clinical status represents a partial response. The complete disappearance of all contrast-enhancing lesions on T1-weighted scans, with stable or improved size of T2-signal lesions, no new lesions, absence of corticosteroid therapy, and stable or improved clinical status, all for a minimum of 4 weeks, represents a complete response. All other results are considered to represent stable disease. For patients who show a response to therapy in the context of a clinical trial, the patient’s best response (i.e., the nadir of the target lesion volume on serial follow-up MRI scans as compared with the baseline scan) is generally recorded for purposes of evaluating therapeutic effect and for any subsequent determination of disease progression. In cases of gross total resection of gadolinium-enhancing disease seen on T1-weighted MRI, only stable disease and disease progression are possible outcomes on subsequent follow-up MRI scans.
the RANO criteria. If there are multiple measurable lesions, then at least two lesions are measured, the products of these dimensions are summed, and the sum total of area-product measurements is taken to represent the burden of residual enhancing tumor. In this context, an increase of gadolinium-enhancing disease on T1-weighted scans by at least 25%, the appearance of any new lesions, the clear progression of nonmeasurable disease, a clear increase in size of T2/fluid-attenuated inversion recovery (FLAIR) signal lesions, or a clear decline in clinical status represents progressive disease. A decrease in gadolinium-enhancing disease on T1-weighted scans by at least 50%, with stable size of T2/FLAIR signal abnormality, no new lesions, stable or decreasing corticosteroid therapy, and stable or improved clinical status represents a partial response. The complete disappearance of all contrast-enhancing lesions on T1-weighted scans, with stable or improved size of T2-signal lesions, no new lesions, absence of corticosteroid therapy, and stable or improved clinical status, all for a minimum of 4 weeks, represents a complete response. All other results are considered to represent stable disease. For patients who show a response to therapy in the context of a clinical trial, the patient’s best response (i.e., the nadir of the target lesion volume on serial follow-up MRI scans as compared with the baseline scan) is generally recorded for purposes of evaluating therapeutic effect and for any subsequent determination of disease progression. In cases of gross total resection of gadolinium-enhancing disease seen on T1-weighted MRI, only stable disease and disease progression are possible outcomes on subsequent follow-up MRI scans.
TABLE 59.1 SUMMARY of THE PROPOSED RANO RESPONSE CRITERIA | ||||||||||||||||||||||||||||||||||||||||
---|---|---|---|---|---|---|---|---|---|---|---|---|---|---|---|---|---|---|---|---|---|---|---|---|---|---|---|---|---|---|---|---|---|---|---|---|---|---|---|---|
|
The RANO criteria’s reliance on conventional anatomic gadolinium enhancement as a surrogate marker for disease response presents a few challenges, because window and level settings can affect the perceived size of a lesion and may differ between readers and viewing settings. In addition, it is well known that postradiation treatment effects can mimic tumor recurrence on contrast-enhanced MRI.30
Pseudo-Progression
In the early-delayed postradiation period—typically defined as the first 6 months after completion of involved field radiotherapy—the appearance of a new or enlarging contrast-enhancing lesion at the site of prior tumor surgery within the high-dose radiation field that spontaneously subsides over serial MRI scans without change in chemotherapy or initiation of new therapy has been termed the radiologic phenomenon of pseudo-progression (Fig. 59.4). This phenomenon has also been defined by histopathology as the presence of necrosis and absence of tumor on tissue specimens obtained through re-operation34 and is most likely induced by pronounced local tissue reaction with a superimposed inflammatory component, edema, and abnormal vessel permeability.35 In recognition by the RANO criteria authors that pseudo-progression is most often observed within the first 12 weeks after radiation therapy completion, true progressive disease can only be designated during these 12 weeks if confirmed by tissue biopsy or by the occurrence of a new measurable lesion outside the high-dose radiation field.33
Pseudo-Response
Another special case arises wherein the patient undergoes antiangiogenic treatment, which can result in a decrease or disappearance of gadolinium-enhancement on conventional anatomic gadolinium-enhanced MRI despite the continued presence of residual or recurrent tumor (i.e., pseudo-response) (Fig. 59.5). In such cases, the unequivocal
progression of disease by T2/FLAIR imaging can confirm progressive disease.33 Although the RANO authors recognized that a variety of promising new imaging techniques (including MR perfusion) have been developed and are currently in use at a growing number of centers, they concluded that none of these techniques have been adequately validated through prospective multicenter imaging trials, and therefore they play no role in the RANO criteria for posttreatment response assessment at this time.33
progression of disease by T2/FLAIR imaging can confirm progressive disease.33 Although the RANO authors recognized that a variety of promising new imaging techniques (including MR perfusion) have been developed and are currently in use at a growing number of centers, they concluded that none of these techniques have been adequately validated through prospective multicenter imaging trials, and therefore they play no role in the RANO criteria for posttreatment response assessment at this time.33
In the late-delayed postradiation period, typically defined as 9 to 12 months and thereafter, the presence of gadolinium-enhancement mimicking tumor recurrence or progression within the postradiation field can occur in radionecrosis (Fig. 59.6).30 Although radionecrosis has been occasionally described as a progressive and irreversible condition, transient gadolinium-enhancement with surrounding edematous T2-signal abnormality has been observed, for example, in humans who have undergone radiation therapy for nasopharyngeal carcinoma, where the brain tissue in question has been exposed to high doses of radiation without underlying tumor involvement.36 Radionecrosis that mimics a high-grade glioma can also be observed following stereotactic radiosurgery for treatment of nontumor disease entities (Fig. 59.7). The presence of radionecrosis may be confirmed by histopathology from reoperation, or it may be confirmed through clinical and radiologic follow-up as in cases of long-term survivors with previously irradiated highly malignant brain tumors such as glioblastoma and gliosarcoma (Fig. 59.8). In practice, there is nearly always a mix of residual tumor and posttreatment effects—including regions of necrosis—observed by pathologists in previously irradiated tissue specimens. The current WHO classification of CNS tumors does not allow for assignment of a WHO tumor grade in such cases. As a result, many pathologists interpret the presence of microvascular proliferation forming glomeruloid structures to be confirmation of an actively growing recurrent neoplasm, and therefore MR perfusion is theoretically an ideal imaging technique for identification of recurrent tumor. It has therefore been suggested to provide imaging biomarkers that correlate with patient outcome in recurrent high-grade glioma.37
Scope of Radiation Injury Suffered and Altered Pattern by Radiation and Chemotherapy Treatment
Radiation necrosis is a severe local tissue reaction to radiotherapy, which can occur as the result of vascular injury, glial and white matter damage, effects on the fibrinolytic enzyme system, or immune mechanisms. The endothelium is particularly susceptible to radiation damage, resulting in greater disruption of the BBB at the site of maximum radiation dose. The reported incidence of radiation necrosis following radiotherapy for brain tumors ranges from 3% to 24%38 and generally occurs 3 to 12 months after radiotherapy but can occur
up to years and even decades later.39 Typical MRI features of radiation necrosis are a “soap bubble” or “Swiss cheese–like” interior of the enhancing lesion that occurs secondary to increased BBB disruption and greater vulnerability to ischemic effects.30 An increase in size of the contrast-enhancing lesion (which mimics tumor progression) followed by subsequent stabilization or resolution of size increase is a relatively common finding seen in up to 20% of patients following concomitant radiotherapy/temozolomide.38 This is termed pseudo-progression, as described previously, and is often observed in the first 2 to 6 months following chemoradiotherapy, with a median of approximately 3 months. Decreased rCBV is seen in both radiation necrosis and in pseudo-progression compared with normal tissue and is also lower than in primary and recurrent tumors. Pseudo-response, on the other hand, is typically identified in patients treated with antiangiogenic agents such as bevacizumab (an anti-vascular endothelial growth factor [VEGF] antibody) and cediranib (a VEGF receptor tyrosine kinase inhibitor). These antiangiogenic agents produce a rapid decrease in contrast enhancement with a high response rate and 6-month progression-free survival (PFS) but with rather modest effects on overall survival (OS).40,41 Previous studies have shown that early decrease in Ktrans (transfer constant) and increase of perfusion metrics such as cerebral blood volume (CBV) and cerebral blood flow (CBF) compared with the pretreatment value are associated with improved PFS and OS.42,43
up to years and even decades later.39 Typical MRI features of radiation necrosis are a “soap bubble” or “Swiss cheese–like” interior of the enhancing lesion that occurs secondary to increased BBB disruption and greater vulnerability to ischemic effects.30 An increase in size of the contrast-enhancing lesion (which mimics tumor progression) followed by subsequent stabilization or resolution of size increase is a relatively common finding seen in up to 20% of patients following concomitant radiotherapy/temozolomide.38 This is termed pseudo-progression, as described previously, and is often observed in the first 2 to 6 months following chemoradiotherapy, with a median of approximately 3 months. Decreased rCBV is seen in both radiation necrosis and in pseudo-progression compared with normal tissue and is also lower than in primary and recurrent tumors. Pseudo-response, on the other hand, is typically identified in patients treated with antiangiogenic agents such as bevacizumab (an anti-vascular endothelial growth factor [VEGF] antibody) and cediranib (a VEGF receptor tyrosine kinase inhibitor). These antiangiogenic agents produce a rapid decrease in contrast enhancement with a high response rate and 6-month progression-free survival (PFS) but with rather modest effects on overall survival (OS).40,41 Previous studies have shown that early decrease in Ktrans (transfer constant) and increase of perfusion metrics such as cerebral blood volume (CBV) and cerebral blood flow (CBF) compared with the pretreatment value are associated with improved PFS and OS.42,43
Differing degrees of neurotoxicity represent a significant clinical complication following brain tumor radio- and chemotherapy. Although a patient may present with acute or subacute clinical symptoms, delayed neurotoxicity manifesting as neurologic symptoms such as neurocognitive dysfunction, white matter degeneration, and possible radiation necrosis is far worse. Recent studies have demonstrated that both high total dosage and individual high-fractionated doses are associated with neurocognitive decline, particularly impairing memory function.44 It has also been established that neurotoxicity is a significant complication following radiation therapy for brain tumors. The radiation injury to the cerebral tissue is complex and involves damage to the vasculature that might be associated with late white matter degeneration or late neurologic symptoms and cognitive dysfunction. Therefore, early changes in cerebral microvasculature may potentially predict future neurocognitive deficits and act as a potential biomarker of radiation injury. Such changes can be studied by perfusion MRI or perfusion CT, as suggested by a recent animal study using dynamic contrast-enhanced and blood oxygenation–level dependent (BOLD) MRI demonstrating a significant increase in Ktrans and reduced BOLD response to carbogen in irradiated regions of brain tumors and reduced microvascular density compared with nonirradiated regions.45 These results suggest that both dynamic contrast-enhanced (DCE) and BOLD MRI techniques can potentially serve as noninvasive biomarkers to detect intratumoral response to radiation therapy. Cao et al.46 have recently shown that early changes in cerebral vasculature can predict cognitive dysfunction secondary to brain irradiation in the form of delayed alterations in verbal learning and total recall. In this study of 10 patients undergoing partial brain radiation therapy (RT) and serial MRI examinations with DCE acquisitions, these authors demonstrated that vascular volumes and BBB permeability increased significantly in the high-dose regions during RT and that these changes were correlated with the cumulative dosage at the time of the MRI scans at weeks 3 and 6 during RT and 1 month following RT. In addition, changes in verbal learning scores 6 months after RT were significantly correlated with changes in the vascular volumes of left temporal and frontal lobes (p <.02, p <.03, respectively), and in changes in BBB permeability of left frontal lobes during RT (p <.007), suggesting that early changes in cerebral vasculature can predict delayed alterations in verbal learning and total recall, important components of neurocognitive function.
Differentiating Recurrent Metastatic Tumor from Radiation Necrosis
Stereotactic radiosurgery is frequently used for treatment of brain metastases, either alone or in combination with whole-brain radiation therapy or surgical resection. Because of the potential for adverse effects from whole-brain radiation therapy in long-term survivors,47 many centers routinely use stereotactic radiosurgery alone for treatment of patients with solitary or limited numbers of brain metastases, reserving whole-brain radiotherapy for those with numerous metastases, leptomeningeal involvement, or diffuse recurrence at the site of prior surgery.48 New metastatic lesions can frequently develop after treatment with stereotactic radiosurgery alone, particularly in those with three or more metastases at the time of initial treatment and those with cancer diagnosis other than non–small cell lung carcinoma, therefore, close follow-up with serial MRI scans may be warranted as reirradiation with stereotactic radiosurgery in such cases can be more effective when new brain metastases are detected early.48,49 Stereotactic radiosurgery can also effectively and safely treat recurrent cerebral metastatic disease after whole-brain radiation therapy has been given,50 regardless of whether the recurrence takes the form of a solitary metastasis or multiple lesions.51
For stereotactic radiosurgery treatment planning of cerebral metastases, thin slice three-dimensional volumetric postgadolinum T1-weighted imaging detects more lesions in approximately one-third of patients as compared with conventional gadolinium-enhanced two-dimensional spin echo MRI sequences obtained at presentation.52 It has also been suggested that 3T MRI using thin slice postgadolinium T1-weighted three-dimensional spoiled gradient echo (SPGR) may enable detection of a greater number of brain metastases than 1.5T MRI using a similar pulse sequence,53 although the degree of enhancement can vary by sequence and field strength (Fig. 59.9). For stereotactic radiosurgery that follows surgical resection of brain metastases, it has been shown that postresection cavities can change in size during the interval between the immediate postoperative MRI scan and the stereotactic radiosurgery treatment planning scan, with up to one-third of cavities becoming larger while the majority do not collapse down to present a smaller treatment target; therefore, acquisition of the stereotactic radiosurgery treatment planning scan as close to the timing of actual treatment is advised, whereas delaying treatment after surgery for the potential benefit of cavity collapse is not.54
After stereotactic radiosurgery has been performed, serial follow-up MRI scans should include the same (or very similar) thin slice three-dimensional T1-weighted gadolinium-enhanced volumetric sequence as was used for treatment planning. Doing so allows direct comparisons to be made between scans, both for accurate treatment response assessment and for early detection of new brain metastases. on serial conventional MRI follow-up scans, most treated metastatic lesions remain stable or decrease in size after stereotactic radiosurgery, while
approximately one-third of lesions may show a transient increase in size, typically beginning at 6 weeks and lasting potentially longer than 15 months.55 An increase in target lesion size after stereotactic radiosurgery can represent tumor relapse, postradiation treatment effect, or a mixture of both.
approximately one-third of lesions may show a transient increase in size, typically beginning at 6 weeks and lasting potentially longer than 15 months.55 An increase in target lesion size after stereotactic radiosurgery can represent tumor relapse, postradiation treatment effect, or a mixture of both.
One approach for distinguishing between recurrent tumor and postradiation treatment effect after stereotactic radiosurgery is to use conventional anatomic gadolinium-enhanced MRI sequences that are obtained for routine posttreatment surveillance. Although some conventional contrast-enhanced MRI features have been reported to suggest the presence of radionecrosis (e.g., ill-defined contrast enhancement with relative absence of mass effect, and a “Swiss cheese” or “soap bubble” pattern of enhancement),30 other authors have found such features to be unreliable in their own reported series and instead have suggested a more quantitative approach by comparing the area of gadolinium enhancement on T1-weighted imaging with the nodular lesion size on T2-weighted imaging.56 Thus a parameter, called the lesion quotient, may be defined as the ratio of nodule size on T2 to the enhancing lesion size on T1 (albeit subject to some measurement variability, as no preset window and level settings have been defined). In their radiographic-pathologic correlation study (n = 32), these authors reported that a lesion quotient of 0.6 or higher was observed in all cases of recurrent tumor (n = 7), while a lesion quotient of 0.3 or less was seen in four of five cases of radionecrosis; of the remaining 20 cases that showed mixed pathology, 19 had a lesion quotient greater than 0.3.56 However, another group found in their own radiologic-pathologic correlation series (n = 51), consisting of tumor progression (n = 33), radiation necrosis (n = 13), and mixed radionecrosis and tumor (n = 5), that the lesion quotient method does not reliably distinguish between tumor recurrence and radiation necrosis, and therefore additional imaging methods are warranted.57 Interestingly, a similar but more qualitative approach called T1/T2 matching was used in another imaging-histopathologic correlation study to show that a correspondence (“T1/T2 match”) between T1-weighted contrast-enhanced volume and the low signal-defined T2-weighted lesion margin was strongly associated with tumor progression, whereas lack of a clear and defined lesion margin on T2-weighted images compared with the margin of T1-weighted gadolinium enhancement (“T1/T2 mismatch”) was strongly associated with postradiation treatment effect and had a sensitivity and specificity of 83.3% and 91.1%, respectively, for identification of radiation effects.58
Among a variety of functional MRI techniques that have been suggested for distinguishing between metastatic tumor recurrence and postradiation, dynamic susceptibility contrast (DSC) perfusion-weighted imaging (PWI) is perhaps the most robust and widely studied. Although technical details vary among institutions and manufacturer platforms, the basic approach has been to acquire serial T2/T2* imaging during the first pass phase of enhancement following a rapid (e.g., 5 cc/s) intravenous 0.1 mmol/kg bolus of gadolinium-based contrast agent followed by a chasing bolus of saline. From relative cerebral blood volume parametric maps generated on a voxel-by-voxel basis from the dynamic imaging data, regions of interest (ROI) are placed around the enhancing lesion and typically within the contralateral normal appearing white matter to calculate a relative CBV normalized ratio (tumor/contralateral white matter). Using this technique at 1.5T after a 0.05 mmol/kg preload of contrast agent (to minimize the effect of T1 shortening from leakage within enhancing lesions),
Mitsuya et al.59 found that a rCBV ratio of greater than 2.1 yielded the best sensitivity and specificity (100% and 95.2%, respectively) for identifying recurrent metastatic tumors in their series (n = 28 lesions in 27 patients; 21 radiation necrosis, 7 recurrent tumor). Using a similar technique without any preload of contrast agent, Hoefnagels et al.60 compared normalized ratios of rCBV to both contralateral gray matter and contralateral white matter (n = 34 lesions in 31 patients; 20 tumor recurrence, 14 tumor necrosis); they found that a cutoff value of 1.85 for maximal tumor CBV relative to normal gray matter improved specificity to 100% over using a threshold of 2.0 when normalizing to white matter (specificity 71.4%), whereas the sensitivity decreased to 70.0% from 85% when normalizing to gray matter rather than white matter. Barajas et al.61 reported similar results (sensitivity 91.3%, specificity 72.7%) using maximal tumor rCBV normalized to contralateral white matter, although their optimal cutoff value was lower (1.54) in their series (n = 30 lesions in 27 patients; 20 recurrent metastases, 10 radiation necrosis without viable tumor). They also found that a higher percentage of signal intensity recovery within contrast-enhancing regions was found in cases of radiation necrosis, and that a cutoff value of greater than 76.3% for percentage of signal intensity recovery yielded a sensitivity of 95.7% and a specificity of 100% for identification of radiation necrosis (although also performed without compensation for leakage effects).
Mitsuya et al.59 found that a rCBV ratio of greater than 2.1 yielded the best sensitivity and specificity (100% and 95.2%, respectively) for identifying recurrent metastatic tumors in their series (n = 28 lesions in 27 patients; 21 radiation necrosis, 7 recurrent tumor). Using a similar technique without any preload of contrast agent, Hoefnagels et al.60 compared normalized ratios of rCBV to both contralateral gray matter and contralateral white matter (n = 34 lesions in 31 patients; 20 tumor recurrence, 14 tumor necrosis); they found that a cutoff value of 1.85 for maximal tumor CBV relative to normal gray matter improved specificity to 100% over using a threshold of 2.0 when normalizing to white matter (specificity 71.4%), whereas the sensitivity decreased to 70.0% from 85% when normalizing to gray matter rather than white matter. Barajas et al.61 reported similar results (sensitivity 91.3%, specificity 72.7%) using maximal tumor rCBV normalized to contralateral white matter, although their optimal cutoff value was lower (1.54) in their series (n = 30 lesions in 27 patients; 20 recurrent metastases, 10 radiation necrosis without viable tumor). They also found that a higher percentage of signal intensity recovery within contrast-enhancing regions was found in cases of radiation necrosis, and that a cutoff value of greater than 76.3% for percentage of signal intensity recovery yielded a sensitivity of 95.7% and a specificity of 100% for identification of radiation necrosis (although also performed without compensation for leakage effects).
Apart from perfusion MRI, a variety of other functional MRI techniques have been suggested for distinguishing between tumor recurrence and postradiation treatment effects following stereotactic radiosurgery, including diffusion MRI techniques and MR spectroscopy.62,63,64,65,66,67 A range of PET imaging tracers have also been studied for their ability to distinguish tumor recurrence from postradiation injury in the brain.68,69,70,71,72,73 In clinical practice, several of these techniques may be deployed simultaneously, sometimes with varying results (Fig. 59.10). Although an in-depth discussion of each such modality is beyond the scope of this chapter, it is worth remembering that much of the evidence in support of these functional MRI and positron emission tomography (PET) imaging techniques comes from small case series at single institutions, and therefore further validation of these techniques—including MR perfusion—is needed.
Predicting Short-Term Outcome
The prognosis of especially high-grade gliomas in the brain remains poor, despite improvements in surgery, radiation therapy, and chemotherapy. Different prognostic factor analyses have been applied in predicting outcomes in patients with newly diagnosed malignant gliomas. Despite several limitations, such as tumor heterogeneity and inaccurate grading owing to sampling error, for example, histologic tumor type and WHO grade remain the most important prognostic factors. Attempts using imaging criteria, different imaging methods, and novel analyses have been made to support the prognostic information obtained by histologic classification and grading of gliomas. Perfusion-weighted MRI, among other noninvasive imaging methods, has been used in a combined approach with histopathology to help deliver prognostic information.74,75,76,77,78,79 Recently, it has been shown that rCBV might be able to distinguish the stable gliomas from the rapidly progressing gliomas among patients with the same histopathologic grade (WHO grade II).80 In addition, perfusion-weighted imaging techniques have demonstrated the potential to better predict time to progression, clinical outcome, and survival in traditional chemoradiotherapy-treated gliomas75,76,77 as well as in patients treated with novel antiangiogenic therapy.
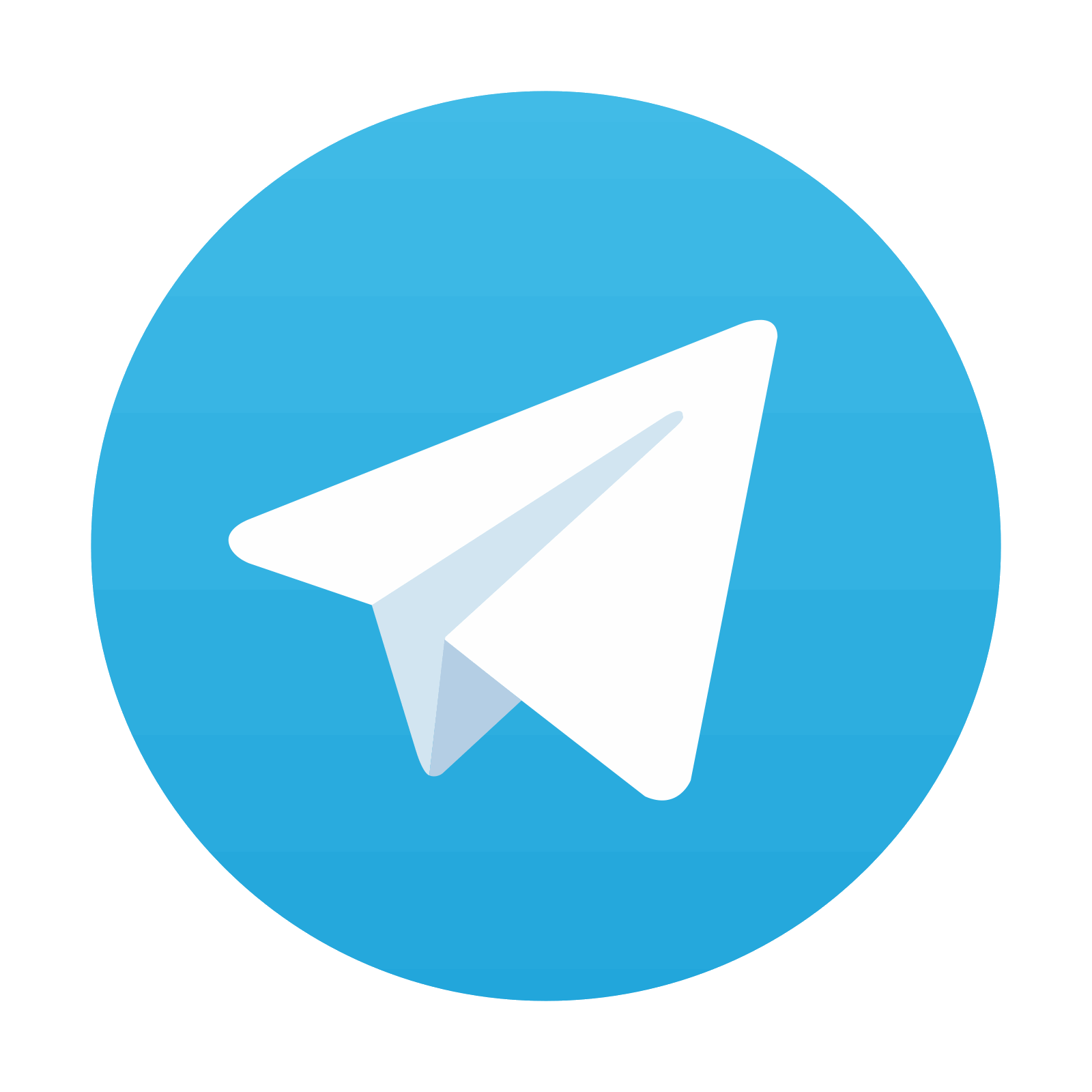
Stay updated, free articles. Join our Telegram channel
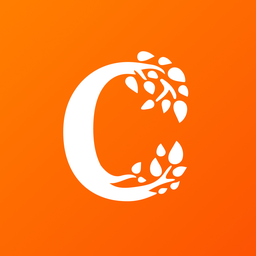
Full access? Get Clinical Tree
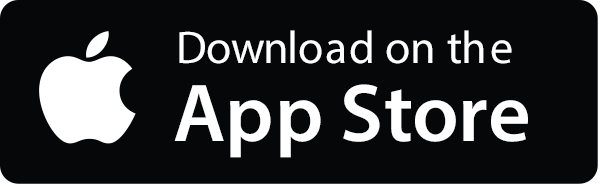
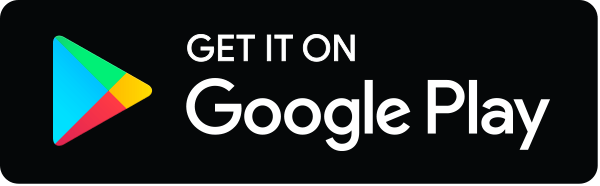