iodinated contrast within a large organ (e.g., brain, heart) can be captured at the same instant in time; this is called isotemporal imaging. Isotemporal imaging is a key feature for accurate CT perfusion analysis in which contrast uptake and washout are imaged at multiple time points. As one could imagine, without isotemporal imaging, it is nearly impossible to separate the change in contrast due to the difference in acquisition time from the change in contrast due to physiologic processes. CT perfusion applications using volume CT are vast and range from neuro applications to tumor or wholeorgan perfusion in the body.20,21,22,23,24,25,26 and 27 With isotemporal resolution, the contrast in the vessels and the surrounding tissues of an organ can be visualized at multiple time points to create a “movie” of the contrast uptake and wash out. This dynamic information can then be used to assess timing of contrast arrival and effect on surrounding tissues. Compared to the helical shuttle technique (see below), volume CT perfusion scanning is lower dose because there is no excess exposure from helical overranging. Helical overranging is the exposure at the beginning and end of a helical run.28 Some of the overranging is necessary for helical reconstruction and some of it is excess. The excess exposure is often mediated with adaptive collimation, but the remaining overranging is unavoidable for helical reconstruction.
dual-source CT was modified to acquire 2 × 128 slices, the gantry rotation time was reduced to 280 milliseconds, and the second tube FOV was increased to 33 cm (Fig. 2.2B). The main goal of creating this scanner was to improve the quality of cardiac imaging by increasing the temporal resolution.30 This construction led to a reduction of the rotation needed to acquire the required projection data for image reconstruction from half of a rotation in single-source CT to a quarter of a rotation in dual-source CT using a monosegment reconstruction mode that is consistent throughout various heart rates owing to individual adaptation of the table pitch.31 Another desirable approach in the new generation of the scanner has been widening of the scan field for the second tube/detector system to improve coverage for other applications, especially perfusion imaging and dual-energy scanning. This required decreasing gantry rotation speed in order to maintain the temporal resolution for cardiac scanning and a system angle of slightly larger than 90 degrees between the two tube-detector systems (Fig. 2.2B). With this new geometry, the two separate x-ray tube and detector array systems are arranged 94 degrees apart, which also allows helical data acquisition at a high pitch. In contrast to the single-source multidetector CT (MDCT), where the maximum pitch is roughly 1.5 in order to reconstruct gapless images, the second-generation dual-source CT can achieve gapless z-axis sampling even at a pitch value of up to 3.2.32 Therefore, it enables coverage of the entire heart in a single heartbeat. However, slow and regular heart rates are required for this single-heartbeat acquisition, which uses prospectively triggered ECG gating. For heart rates greater than 55 or 60 beats per minute, multiphase acquisition is used, which requires pitch ranges from 0.2 to 0.5 (depending on the heart rate), and a retrospective ECG gating will be used for data acquisition.33,34 Due to the high temporal resolution, the scanner has been commonly used for the assessment of coronary artery stenosis and is currently being assessed for myocardial perfusion.35,36 Another application of dualsource CT is tissue characterization when both detector systems are operated at different kilovolts, so-called “dual-energy CT.”37 One limitation of this scanner particularly in perfusion studies has been small detector span to cover the entire anatomy of interest in one pass. This limitation has been partially solved using dynamic spiral scanning with variable pitch (“toggling table” technique).38 One problem with the anatomic configuration of dual-source scanners is image degradation due to the presence of scatter radiation induction in the presence of two tubes. To solve this issue, special scattered radiation correction algorithms are used. The mounted x-ray tubes in dual-source scanners are also smaller and lighter than traditional x-ray tubes, which have larger anodes rotating in a vacuum. To improve heat capacity of the small tubes, the anode is placed in direct contact with cooling oil. When both tubes are working, the radiation dose will be doubled. To avoid this problem, lowering dose strategies have been implemented including, 3D adaptive noise reduction filter (while preserving edge detail), iterative reconstructions, heart rate-dependent pitch values, and ECG-based tube current modulation. A cardiac or body beam-shaping filter (bowtie or wedge filter) and small FOV can be used to attenuate or eliminate unnecessary radiation to the body periphery.
low- and high-energy spectra (e.g., 80 and 140 kVp) will be decomposed into two basis materials such as water and iodine to form the material density pair. Alternatively, DECT data can be decomposed into energy functions and can be used to generate monochromatic images through sophisticated reconstruction algorithms42 (Fig. 2.5). For image-based methods, the dual-energy data processing is performed after the reconstruction of high- and low-energy images (image-based domain). DECT images are created through various mathematical combinations of the low-energy and high-energy images such as subtraction and blending. Monochromatic spectral image processing is not possible with image-based dual-energy methods, only with raw data-based methods. Currently, CT systems from most vendors are capable of dual-energy material decomposition. Some of these systems are also capable of raw data-based
monoenergetic CT image reconstruction. There are three different CT designs to perform dual-energy decomposition (Table 2.1):
Single source with rapid kVp switching (Fig. 2.6A and B).
Dual-source scan in that high- and low-energy beams are produced during one scan (Fig. 2.7).
Single source using sandwich detectors or energy-discriminating photon-counting detectors (Fig. 2.6C). The latter method uses one kVp setting and detects radiation using innovative, spectral CT detectors that separate the x-ray beam into its spectral components (i.e., polychromatic x-ray detection).
TABLE 2.1 Dual-Energy CT Techniques | ||||||||||||||||
---|---|---|---|---|---|---|---|---|---|---|---|---|---|---|---|---|
|
between kVps, which is optimal for reducing patient motion between kVp settings. No tube current modulation can be used (limiting dose reduction options), and the same mA must be used for both kVp levels. Since the mA is maintained at a constant value for both kVp levels, it is necessary to run the DECT mode at an mA level that is low enough to minimize excess exposure from the 140-kVp beam while ensuring it is high enough to obtain the necessary image quality from the 80 kVp. As a result, the 80-kVp images may be too noisy, or the 140-kVp images may be generated with unnecessarily high dose.
save radiation, improving lesion conspicuity, iodine extraction, and improving tissue/material characterization (e.g., renal stone composition).49,50 and 51 In angiographic studies, higher intravascular attenuation on 50- and 70-keV monochromatic images can potentially enable high-quality CT angiography (CTA) studies at lower doses of contrast media.52,53 and 54 The use of colored iodine overlay to improve the detection of endoleaks and the subtraction of heavily calcified plaques to improve visualization of vessel stenosis are additional clinical applications. While it is relatively easy to separate iodine in wellopacified vessels from dense calcium in cortical bone or large calcified atherosclerotic plaques, this may not be the case when the density of contrast and calcium is in the same range. It is also technically challenging to separate calcified plaques in small coronary arteries.
factors may be necessary to compensate for photon starvation and decreased SNR. In MDCT, noise increases if pitch is greater than 1 (assuming technique factors are held constant). When pitch is greater than 1, photon-deficient zones can occur in the z-direction causing increasing noise. This phenomenon is less apparent for studies using pitch less than 1 (e.g., cardiac).60 In most scanners, tube current-time product is automatically increased in proportion to increasing pitch to keep noise level low. The term “effective” mAs is used by Siemens and equals to mAs/pitch in noncardiac scan modes, and in cardiac mode, the term total mAs delivered per gantry rotation is used, which is defined as mAs/rotation.
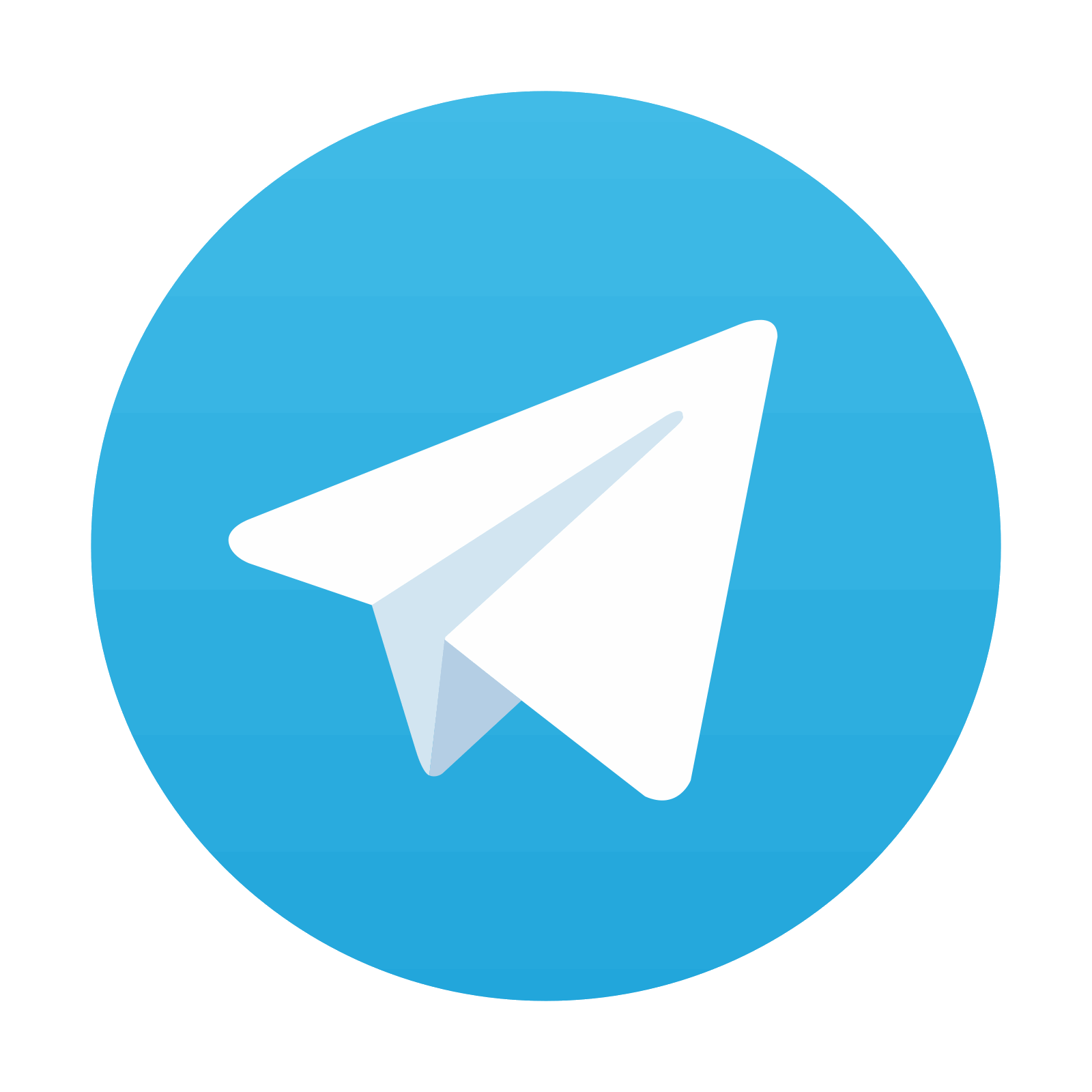
Stay updated, free articles. Join our Telegram channel
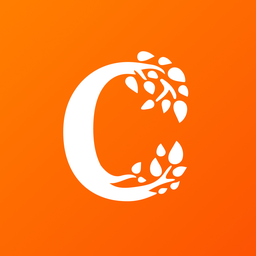
Full access? Get Clinical Tree
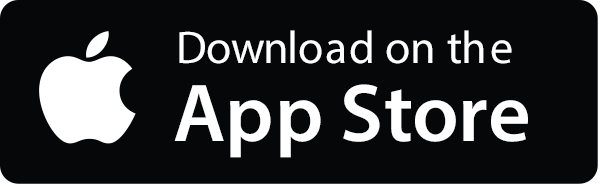
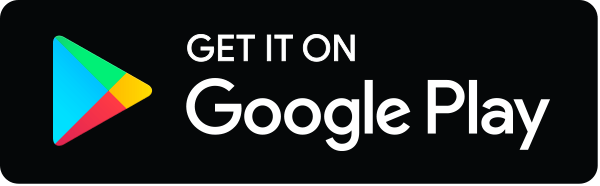