In this article, the basics of diffusion-weighted imaging/diffusion tensor imaging (DTI) are discussed, including a short historical perspective on the fiber dissection technique, followed by a review of selected brain malformations in which DTI and tractography have contributed to a better understanding of the malformations, and by a clinical case in which DTI showed a disorder of the internal neuroarchitecture that could not be correctly appreciated by conventional anatomic magnetic resonance imaging.
Key points
- •
Diffusion tensor imaging (DTI) is an advanced magnetic resonance (MR) technique that provides qualitative and quantitative information about the microarchitecture of white matter.
- •
DTI may show important information about the brain microstructure in brain malformations that may go undetected or remains underestimated on conventional MR sequences.
- •
DTI may better categorize various brain malformations that may look similar on conventional MR imaging, but may be caused by different pathomechanisms.
- •
Human disorders of axonal guidance result from aberrant axonal wiring and are caused by mutations in genes that code for molecules that guide axons within the brain.
- •
Much of our knowledge about human disorders of axonal guidance comes from DTI studies of crossing tracts, such as the corpus callosum, optic chiasm, corticospinal tract, and cerebellar peduncles.
Introduction
Diffusion-weighted imaging (DWI) and in particular diffusion tensor imaging (DTI) have propelled our noninvasive exploration and understanding of many common and rare congenital brain abnormalities, which include malformations as well as disruptions. DWI/DTI takes advantage of the preferential mobility and diffusion of water molecules in three-dimensional space within biological tissues with a complex microstructural architecture like the brain. The mobility of water molecules in the brain is dependent on multiple factors, including the microstructural architecture, which is believed to be predominantly determined by the alignment and diameter of fiber tracts and their myelin sheets as well as the three-dimensional distribution and arrangement of neurons. In addition, multiple other spatially organized flow patterns exist within the brain, like blood flow in the arterial and venous networks and the molecular flow of protons, which depend on active and passive transport mechanisms within the brain. Exploring the differential mobility and diffusion of water molecules in the brain gives indirect information about the normal and abnormal internal architecture, networks, and integrity of the pediatric brain.
The differential, three-dimensional direction and magnitude of water mobility can be measured noninvasively, in vivo by adding diffusion-weighted gradients to standard ultrafast magnetic resonance (MR) sequences. Typically, diffusion gradients are applied along multiple noncollinear directions in space to resolve the complete diffusion tensor. The more diffusion directions gradients measured, the better is the three-dimensional shape and magnitude of the sampled diffusion tensor. Powerful postprocessing tools subsequently allow reconstructing the course of major fiber tracts within the central nervous system (CNS). DTI has been of invaluable significance to explore common and uncommon brain malformations. Steve Jobs, one of the most influential inventors and entrepreneurs of our time, made a quote that summarizes the goal and value of DTI in brain malformations: “because believing that the dots will connect down the road, will give you the confidence to follow your heart, even when it leads you off the well-worn path.” The DTI acquisitions render data sets that have to be postprocessed with an open scientific mind, in which the various dots or seed points have to be connected in a nonbiased mathematical approach to confirm the course of well-known common pathways and networks, which should also allow us to explore and discover unknown or aberrant fiber tracts and anatomic connections. Recognizing these aberrant pathways may be challenging but help us to better understand, classify, or group brain malformations. This approach is similar to high-end brain teasers, in which numbers need to be discovered using a complex mathematical formula and then connected to uncover or recognize a hidden figure ( Fig. 1 ).
In this article, the basics of DWI/DTI are discussed, including a short historical perspective on the fiber dissection technique, followed by a review of selected brain malformations in which DTI and tractography have contributed to a better understanding of the malformations, and by a clinical case in which DTI showed a disorder of the internal neuroarchitecture that could not be correctly appreciated by conventional anatomic MR imaging.
Introduction
Diffusion-weighted imaging (DWI) and in particular diffusion tensor imaging (DTI) have propelled our noninvasive exploration and understanding of many common and rare congenital brain abnormalities, which include malformations as well as disruptions. DWI/DTI takes advantage of the preferential mobility and diffusion of water molecules in three-dimensional space within biological tissues with a complex microstructural architecture like the brain. The mobility of water molecules in the brain is dependent on multiple factors, including the microstructural architecture, which is believed to be predominantly determined by the alignment and diameter of fiber tracts and their myelin sheets as well as the three-dimensional distribution and arrangement of neurons. In addition, multiple other spatially organized flow patterns exist within the brain, like blood flow in the arterial and venous networks and the molecular flow of protons, which depend on active and passive transport mechanisms within the brain. Exploring the differential mobility and diffusion of water molecules in the brain gives indirect information about the normal and abnormal internal architecture, networks, and integrity of the pediatric brain.
The differential, three-dimensional direction and magnitude of water mobility can be measured noninvasively, in vivo by adding diffusion-weighted gradients to standard ultrafast magnetic resonance (MR) sequences. Typically, diffusion gradients are applied along multiple noncollinear directions in space to resolve the complete diffusion tensor. The more diffusion directions gradients measured, the better is the three-dimensional shape and magnitude of the sampled diffusion tensor. Powerful postprocessing tools subsequently allow reconstructing the course of major fiber tracts within the central nervous system (CNS). DTI has been of invaluable significance to explore common and uncommon brain malformations. Steve Jobs, one of the most influential inventors and entrepreneurs of our time, made a quote that summarizes the goal and value of DTI in brain malformations: “because believing that the dots will connect down the road, will give you the confidence to follow your heart, even when it leads you off the well-worn path.” The DTI acquisitions render data sets that have to be postprocessed with an open scientific mind, in which the various dots or seed points have to be connected in a nonbiased mathematical approach to confirm the course of well-known common pathways and networks, which should also allow us to explore and discover unknown or aberrant fiber tracts and anatomic connections. Recognizing these aberrant pathways may be challenging but help us to better understand, classify, or group brain malformations. This approach is similar to high-end brain teasers, in which numbers need to be discovered using a complex mathematical formula and then connected to uncover or recognize a hidden figure ( Fig. 1 ).
In this article, the basics of DWI/DTI are discussed, including a short historical perspective on the fiber dissection technique, followed by a review of selected brain malformations in which DTI and tractography have contributed to a better understanding of the malformations, and by a clinical case in which DTI showed a disorder of the internal neuroarchitecture that could not be correctly appreciated by conventional anatomic MR imaging.
Basics of diffusion-weighted imaging and diffusion tensor imaging
DWI generates image contrast based on differences in diffusion characteristics of water molecules within the brain. Diffusion represents the random thermal movement of molecules, also known as Brownian motion, within tissues. Diffusion within the brain is determined by a variety of factors, including the type of molecule under investigation, the temperature of the tissue, and most importantly, the microenvironmental architecture in which the diffusion takes place. For example, the rate of diffusion of water molecules within the cerebrospinal fluid (CSF) is higher than the rate of diffusion of water molecules within the brain tissue. By adding diffusion-encoding gradients to standard MR imaging sequences, these differences in diffusion rates can be translated into and shown as differences in MR signal. These differences in MR signal of the individual imaging voxels can be mapped two-dimensionally as so-called DWI images. Typically, isotropic DWI images are generated ( Fig. 2 ). Isotropic DWI images incorporate signal related to both the rate of diffusion and T2-relaxation phenomena. In addition, maps of apparent diffusion can be calculated, also known as apparent diffusion coefficient (ADC). The ADC values are typically displayed as two-dimensional gray-white scale maps, in which the calculated ADC values are translated into brightness maps or so-called ADC maps. Areas with high degrees of diffusion or high ADC values appear bright (eg, CSF), whereas areas with low degrees of diffusion or low ADC values (eg, densely packed white matter tracts) appear darker. The major advantage of ADC is that the T2 contribution of the DWI maps is canceled out, giving a map of the spatial distribution of diffusion in the brain without contamination by the T2-relaxation phenomena (see Fig. 2 ).
DTI takes DWI to the next level of image-based microstructural tissue exploration. The three-dimensional shape and principal direction of diffusion as well as the magnitude of diffusion (diffusion rate) within space differ between the various brain structures. The microstructural architecture as well as physiologic factors determine the diffusion of water molecules within the brain. The effective molecular diffusion in the white matter tracts is, for example, predominantly along the direction parallel to the long axis of white matter tracts and limited in the direction perpendicular to the white matter tracts. The three-dimensional shape of diffusion in white matter tracts resembles an ellipsoid, which is also known as anisotropic diffusion ( Fig. 3 A). When the degree of diffusion is equal in all directions such as in CSF, in which no barriers limit diffusion, the three-dimensional shape of diffusion resembles a sphere, which is also known as isotropic diffusion (see Fig. 3 B). The three-dimensional shape of diffusion can be studied by measuring the full tensor of the diffusion (DTI), in which diffusion gradients are applied along at least 6 noncollinear directions in space. Maps of the spatial distribution and magnitude of the anisotropic component of diffusion are generated. Fractional anisotropy (FA) maps are typically calculated, in which the assigned signal intensity is related to the degree of anisotropic diffusion. FA values range between 0 and 1. An FA value of 0 indicates complete isotropic diffusion, and an FA value of 1 indicates complete anisotropic diffusion ( Fig. 4 ). These FA values can be mapped topographically, in which voxels with an FA value of 0 are black and voxels with an FA value of 1 are white. In addition, the principal direction of diffusion in three-dimensional space can be color coded ( Fig. 5 ) or can be shown as a vector for each voxel ( Fig. 6 ). Blue represents a predominantly craniocaudal diffusion, red represents predominantly left to right diffusion, and green represents predominantly anterior-posterior diffusion within the brain. These maps provide information about the architecture and integrity of organized tissues. Finally, by combining the magnitude and directional information of anisotropic diffusion of the measured voxels, white matter tracts can be calculated/reconstructed. Voxels with a similar orientation and magnitude of their principal anisotropic diffusion direction are likely to be part of the same white matter tract. Powerful postprocessing mathematical algorithms that connect these voxels (or dots, as mentioned in the quote by Steve Jobs) allow white matter tracts to be studied and visualized in vivo (also known as fiber tractography [FT]) ( Fig. 7 ).
White matter exploration from Andreas Vesalius to Josef Klingler and beyond
The recognition of high-order functionality of the CNS, relying on the complex networking of multiple functional centers by white matter tracts, dates back to the early sixteenth century. Observational and anatomic studies performed by scientists like Andreas Vesalius (1514–1564) and René Descartes (1596–1650), who were anatomists, physicians, and authors of 2 of the most influential books on human anatomy, De Humani Corporis Fabrica and De Homine Figures et Latinitate Donates , identified and reported white matter tracts within the CNS. The textbook of Descartes was published posthumously by Florant Schuyl in 1662 in Latin and again in 1664 in French by Claude Clerselier, entitled Le Traité de l’Homme . Illustrations in the textbooks show how, for example, heat from a fire close to the foot is transmitted to the brain via a tract extending from the foot via the spinal cord to the brain ( Fig. 8 ). In addition, several figures show that the eyes are connected to the brain over the optic nerves, which again appear to extend to the occipital lobes. Several additional early descriptions about white matter tracts exist in the literature. Marcello Malpighi (1628–1694) showed in 1669 that the cerebral white matter is composed of fibers, and Niels Stensen (1638–1686) studied the course of white matter tracts in the brain by following the nerve threads through the brain substance. In the decades and centuries to follow, many more discoveries were made based on the detailed evaluation of the cerebral white matter, partially taking advantage of the scraping method for dissecting cerebral white matter, as suggested by Stensen. The scraping technique allowed studying the branching of white matter tracts in better detail, especially after the brain was prepared by various fixation techniques (eg, injection of alcohol).
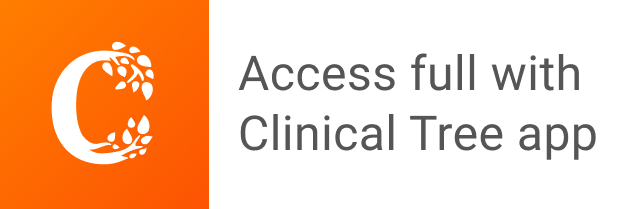