Fig. 13.1
Schematic representation of the EPR effect. Nanoparticles cannot cross tight junctions in normal tissues, but extravasate into tumor tissue through large inter-endothelial gaps in the defective tumor microvasculature
Ultrasound as a component of a drug delivery system may be coupled with a variety of drug carriers. An ideal drug carrier for ultrasound-mediated drug delivery should satisfy a number of requirements: stability in circulation; drug retention until activated; size that allows extravasation through defective tumor vasculature; ultrasound responsiveness.
13.2 Ultrasound Effects in Drug Delivery: Anticipated Mechanisms
Several mechanisms of ultrasound action in drug delivery have been discussed (Dalecki 2004; Deckers and Moonen 2010; Ferrara 2008; Frulio et al. 2010; Miller et al. 1996; Rapoport et al. 2009a, b, Rapoport et al. 2011, 2013); both ultrasound-triggered localized drug release from carriers and effects of ultrasound on biological tissue should be considered.
13.2.1 Thermal Effects
Ultrasound produces localized tissue heating. Tissue heating depends on the tissue absorption of energy and rates of thermal diffusion and convection. Absorption of ultrasound energy is frequency dependent and increases monotonically with frequency. Even a moderate temperature increase may have serious biological consequences, e.g. significantly increase permeability of blood capillaries (Dreher et al. 2006; Kong et al. 2000b, 2001) and/or lead to cell membrane fluidization (Hayat and Friedberg 1986; Krupka et al. 2009). Thermal effects of ultrasound have been used with temperature-sensitive liposomes that rapidly release their contents at physiologically tolerated tissue temperatures (Dewhirst et al. 2005; Gaber et al. 1996; Hauck et al. 2006; Kong et al. 2000a, b; Kong and Dewhirst 1999; Needham et al. 2000; Negussie et al. 2011; Vujaskovic et al. 2010; Yarmolenko et al. 2010). Heating produces a gel-to-fluid phase transition in the phospholipid membrane that enhances release and diffusion of drug in the target region. Doxorubicin-loaded liposomes have been commercialized (ThermoDox®, Celsion Corp.) and are undergoing clinical trials in combination with radiofrequency (RF) thermal ablation (Hauck et al. 2006; Poon and Borys 2009). Ultrasound as a heating modality has also been studied for release of drugs from loaded liposomes, and other similar temperature-sensitive liposomes (Deckers and Moonen 2010; Dromi et al. 2007; Negussie et al. 2011; Staruch et al. 2011; Stone et al. 2007).
13.2.2 Mechanical Action of Ultrasound: Cavitation
Cavitation phenomena may exert substantial effects on biological tissues and drug carriers. Cavitation can be enhanced by the introduction of gas-filled microbubbles. In current clinical practice, microbubbles have been used as ultrasound contrast agents for cardiovascular imaging (Becher and Burns 2000; Becher et al. 2005) and for molecular imaging (Klibanov 2007). During the last decade, microbubbles have attracted attention as drug carriers and enhancers of drug and gene delivery (See Chap. 11). In the ultrasound field, microbubbles grow and collapse in a process of inertial cavitation. Inertial cavitation of microbubbles creates microjets and shock waves that can create holes in blood vessels and cell membranes (sonoporation), thus increasing permeability of biological barriers for drugs, genes and their carriers (see Chaps. 9 and 10). At ultrasound energies that don’t induce inertial cavitation, microbubbles stably oscillate in the ultrasound field. Stable cavitation of systemically injected microbubbles can induce alternating invagination and distention of blood vessel walls, which in turn can damage the endothelial lining and temporarily increase blood vessel permeability (Chen et al. 2010; Chen et al. 2011; Gaitan et al. 2010; Matula and Guan 2011). For blood vessels that are large in comparison to microbubble sizes, invagination appears to be a major vessel damaging factor; for small blood capillaries, both invagination and distension results in endothelial damage and increased permeability (Chen et al. 2011).
Several research groups have concentrated their efforts on developing microbubble-based drug delivery systems (see Chaps. 11 and 12). Microbubble cavitation has been used for enhancing drug delivery from liposomes (Frulio et al. 2010; Hernot and Klibanov 2008; Klibanov et al. 2010). The efficient therapeutic action of microbubbles combined with low duty cycle ultrasound on subcutaneously grown glioma xenografts was reported (Burke et al. 2011).
Microbubble cavitation was successfully used for opening the blood–brain barrier to allow effective delivery of drugs to the brain (see Chap. 16). Drug-loaded microbubbles have also been developed for targeting intravascular targets (see Chaps. 18 and 19), and tissue plasminogen activator (tPA)-loaded microbubbles combined with ultrasound have been developed for thrombolysis (Hitchcock and Holland 2010; Hitchcock et al. 2011; Laing et al. 2011; Shaw et al. 2009; Smith et al. 2010; Sutton et al. 2013a, b). The biological effects of microbubbles are based on the enhanced penetration of various nanoscaled particles or drugs through biological barriers (Mohan and Rapoport 2010; Thakkar et al. 2013).
13.2.3 Mechanical Action of Ultrasound in the Absence of Cavitation
The most frequently discussed non-thermal and non-cavitation mechanism of ultrasound is related to acoustic streaming and ultrasound radiation force. Sound propagating through a medium produces a force upon the medium (acoustic streaming) and the particles suspended in the medium (radiation force) (Dayton et al. 1999, 2006). Acoustic streaming and radiation force each produce particle translation in the acoustic field, and their effects may be combined. It was demonstrated that acoustic streaming and/or radiation force present a means to localize and concentrate particles near a vessel wall, which may assist the delivery of targeted agents. The radiation force pulses can bring a drug carrier into proximity to a cell, which would facilitate adhesion of the carrier or its fragments to cell membranes (Shortencarier et al. 2004). This would be especially effective for enhancing active targeting that is based on ligand/receptor interaction. Actively targeted acoustically active lipospheres were used to deliver paclitaxel (PTX) to HUVEC cells overexpressing ανβ3-integrins (Tartis et al. 2006). Circulating drug-loaded particles were first deflected by radiation force to a vessel wall and were subsequently fragmented by stronger pulses (Dayton et al. 1999). A similar strategy was used for enhancing the cellular interaction of targeted lipid-coated perfluorooctylbromide (PFOB) nanoparticles with melanoma cells (Soman et al. 2006). The authors hypothesized that the ultrasound facilitated drug transport from the perfluorocarbon nanoparticles into cells due to direct cell/nanoparticle interaction that stimulated lipid exchange and drug delivery. Acoustic streaming appears to dominate in large blood vessels (with hundreds micrometers per second for particle displacement), while radiation force is expected to dominate in the microvasculature because acoustic streaming decreases with decreasing vessel diameter (Dayton et al. 2006). Acoustic streaming and radiation force can push nanoparticles through blood capillary walls, thus enhancing extravasation of drug carriers or macromolecular drugs (Dayton et al. 1999, 2006; Ferrara 2008; Holland and McPherson 2009; Stieger et al. 2007; Thakkar et al. 2013).
In addition to cavitation, non-cavitation mechanisms may be effective for microbubble action. For perfluorocarbon microbubbles, the mismatch between acoustic impedances of water or tissue (1.4 MRayl) and perfluorocarbon (approx. 0.3 MRayl) may promote generation of shear stresses. This in turn would increase inter-endothelial gaps and extra-cellular spaces, resulting in increased extravasation and diffusion of drug carriers and drugs in sonicated tissues (Frenkel et al. 2000a, b, 2006; Hancock et al. 2009a, b; O’Neill et al. 2009; Yuh et al. 2005). In an interesting novel application, ultrasound radiation force was used to modulate ligand exposure on the surface of actively targeted contrast agents (Lum et al. 2006).
Ultrasound-triggered localized drug release may be activated using carriers that are sensitive to either mechanical factors, thermal factors, or both (Borden et al. 2005, 2008; Dayton et al. 2006; Ferrara et al. 2007; Ferrara 2008; Gao et al. 2004; Kheirolomoom et al. 2007, 2010; O’Neill and Rapoport 2011; Qin et al. 2009; Rapoport 2012a, b; Schroeder et al. 2007; Schroeder et al. 2009; Unger et al. 2004; Wheatley et al. 2006; Zheng et al. 2008). Ultimately, the combined thermal and mechanical actions of ultrasound on drug carriers and biological tissues enhance perfusion, increase extravasation of drug carriers and drugs, and facilitate drug penetration through other biological barriers. Consequently, this enhances drug diffusion throughout tumor tissue, resulting in significantly enhanced therapeutic efficacy of conventional drugs (see below) (McDannold et al. 2008; McDannold et al. 2006; Rapoport et al. 2009b, 2010b, 2011; Treat et al. 2007; Vykhodtseva et al. 2006, 2008).
The most straightforward and cost-effective way to develop microbubble-based drug delivery systems would be to load drugs into the FDA approved ultrasound contrast agents, such as Optison® (Amersham Inc.) or Definity® (Lanteus Medical Imaging Inc.). While this approach may be very beneficial for targeting intravascular targets, currently used ultrasound contrast agents present a number of inherent problems as interstitial-targeted drug carriers. A very short circulation time (minutes) of commercially available microbubbles, and their large size (2–10 microns), do not allow effective extravasation into tumor tissue, thus preventing effective drug targeting. This problem may be solved by developing nanoscaled phase-shift microbubble precursors, which convert into microbubbles under ultrasound action as discussed below.
13.3 Phase-shift Perfluorocarbon Nanoemulsions as Drug Carriers for Ultrasound-mediated Drug Delivery
As mentioned above, microbubbles as drug carriers present inherent problems for interstitial delivery to tumors. To solve this problem, drug-loaded nanoscaled microbubble precursors that accumulate in tumors via passive or active targeting, which then convert into microbubble in-situ under the action of ultrasound have been developed. The microbubble precursors are perfluorocarbon (PFC) nanoemulsions.
13.3.1 Generation of Perfluorocarbon Nanoemulsions
13.3.1.1 General Approach
Perfluorocarbon micro- and nanodroplets are manufactured by emulsification of a water/surfactant/perfluorocarbon mixture. Various surfactants, perfluorocarbons and emulsification means have been explored. In the works by Fowlkes’s group, dodecafluoropentane (also called perfluoropentane, PFP) was used as a droplet core, with albumin used as a droplet shell; emulsification was performed in a high-speed shaker (Kripfgans et al. 2000). PFP mixtures with a higher boiling temperature than PFC were used by the Ferrara group, with a lipid mixture used for droplet stabilization (Dayton et al. 2006). For preparing polymer-coated perfluorooctyl bromide (PFOB) “nanocapsules”, a poly(lactic-glycolic acid) copolymer was co-dissolved with PFOB in an organic solvent. The mixture was then pre-emulsified in a high-speed shaker and additionally emulsified by ultrasound; organic solvent was removed by evaporation (Reznik et al. 2011). Acoustic characterization of these nanodroplets suggested that vaporization may be induced by diagnostic 7.5 MHz ultrasound at a mechanical index in the diagnostic range (MI <1) (Reznik et al. 2011). In order to decrease droplet sizes, crude emulsions are usually additionally processed through a microfluidizer (Reznik et al. 2014). For delivering water soluble compounds (fluorescein or thrombin), a double emulsion technique has been developed (Fabiilli et al. 2010b).
A new approach for the generation of highly acoustically active perfluorocarbon droplets was recently suggested (Matsunaga et al. 2012; Sheeran et al. 2011). The authors formulated nanodroplets from highly volatile decafluorobutane (DFB) (boiling point about −2 °C). Nanodroplets were stable at physiological temperatures, but were activated by ultrasound in-vivo using pressures within the FDA guidelines for diagnostic imaging (Matsunaga et al. 2012). A different approach to generation of drug-loaded PFC nanodroplets was suggested in the works by Rapoport’s group. Here, drug-loaded polymeric micelles were used as a starting point for generating nanoemulsions (Gao et al. 2008; Rapoport et al. 2010a; Rapoport et al. 2007, Rapoport et al. 2009a, b, 2007, 2010b, 2011). Manufacture simplicity, absence of toxic solvents and increased drug loading capacity are attractive features of this technology. In addition, amphiphilic block copolymer micelles (if they are preserved in a formulation) may exert extremely important biological effects by preventing development of drug resistance (Alakhov et al. 1996; Alakhova et al. 2010; Batrakova et al. 1999, 2001, 2003).
13.3.1.2 Polymeric Micelles as a Starting Point for Generation of Drug-loaded PFC Nanodroplets
Polymeric micelles are formed by self-assembly of individual amphiphilic block copolymer molecules (unimers) in aqueous milieu. Hydrophobic blocks form micelle cores, while hydrophilic blocks (usually PEG) form micelle corona (or shells). PEG shells are important for suppression of nanodroplet uptake by reticuloendothelial system cells. Lipophilic drugs are solubilized in micelle cores. Many different polymeric micelle systems have been designed. The application of biodegradable, pH-sensitive micelles, like those of poly(ethylene oxide)-co-poly(D,L-lactide) (PEG-PDLA) or poly(ethylene oxide)-co-poly(caprolactone) (PEG-PCL), are especially appealing. When internalized by tumor cells via endocytosis, the micelles move to the acidic environment of endosomes and lysosomes, where their hydrolysis at low pH results in drug release. The amphiphilic character of block copolymer micelles, their size (between 20 nm and about 100 nm) and surface properties provide a relatively high drug loading capacity and long circulation time in the vascular system, which is important for effective tumor targeting. However, micelles manifest substantial shortcomings as drug carriers. Micelle formation is thermodynamically driven, meaning micelles dissociate into unimers when copolymer concentration drops below a critical value called the critical micelle concentration (CMC). Systemic injections of micellar formulations are associated with substantial dilutions in the circulatory system. This may result in a premature drug release into circulation before the drug reaches its target, which has presumably occurred in clinical trials. Polymeric micelles as drug carriers are either too unstable, thus prematurely releasing their drug load, or on the contrary too stable, therefore incapable of providing adequate drug release at the tumor site.
A feasible approach to overcoming these complications consists in developing stable micellar systems that could be activated using external triggers (e.g. ultrasound), inducing drug release at a desired body location (Rapoport 2007). This may be done via introduction of some oil, e.g. a PFC compound. At a suitable PFC/copolymer ratio, PFC nanodroplets are formed. Nanodroplets are much more stable against dilution than micelles, and have other important advantages for ultrasound-mediated drug delivery (see below). To produce nanodroplets, a PFC compound was introduced into a micellar solution formed by an amphiphilic block copolymer (i.e. poly(ethylene oxide)-co-poly(L-lactide) (PEG-PLLA), poly(ethylene oxide)-co-poly(D,L-lactide) (PEG-PDLA), or poly(ethylene oxide-co-polycaprolactone (PEG-PCL). The mixture was emulsified on ice under low-frequency ultrasound. A chemotherapeutic drug (Doxorubicin (DOX) or paclitaxel (PTX)) was pre-introduced into a micellar solution (Gao et al. 2008; Rapoport et al. 2007, 2009b, 2011). Perfluorocarbon compounds tested were PFP or perfluoro-15-crown-5-ether (PFCE), however any PFC compound or their mixture may be emulsified this way. PFCE generates more stable and easy to handle nanoemulsions than PFP; in addition, it offers a possibility of monitoring in-vivo nanodroplet biodistribution using 19 F-MRI since twenty equivalent fluorine nuclei in a PFCE molecule generate a sharp peak in MR spectra. A phase state of perfluorocarbon/copolymer formulations depends on the PFC/copolymer concentration ratio as shown schematically in (Fig. 13.2) (Gao et al. 2008). At low concentration, PFC is dissolved in micelle cores (zone 1, Fig. 13.2). When the PFC concentration exceeds a limit of solubility in micelle cores, nanodroplets evolve into a separate phase. In this process, former micelle cores turn into droplet shells.
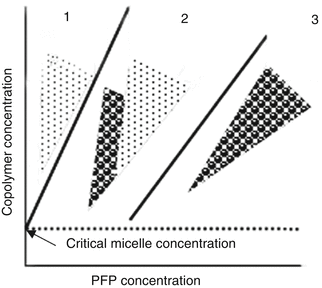
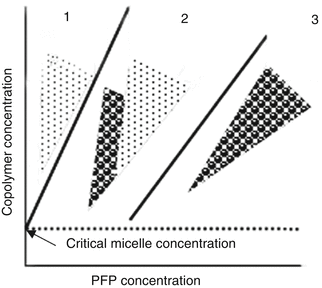
Fig. 13.2
Schematic representation of the phase diagram of a perfluorocarbon/block copolymer formulation in aqueous solution (Reprinted with permission from (Gao et al. 2008))
A nanodroplet shell contains two layers: the inner layer, formed by a hydrophobic block of a block copolymer (e.g. polylactide or polycaprolactone), and the outer layer, formed by a hydrophilic block, usually PEG, as shown schematically in Fig. 13.3a. A lipophilic drug initially encapsulated in micelle cores moves with the hydrophobic block into the inner layer of a droplet shell, as exemplified by the laser confocal imaging of DOX encapsulating droplets (Fig. 13.3b).
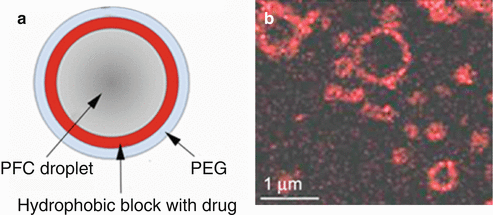
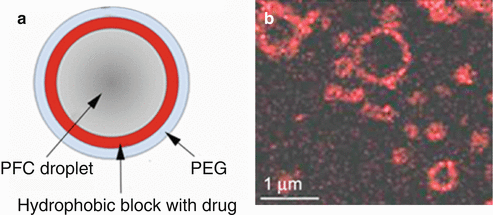
Fig. 13.3
Schematic representation of a drug-loaded nanodroplet (a) and laser confocal image of DOX-loaded PFP/PEG-PLLA droplets; scale bar 1 μm (b); micron-sized droplets were produced to better visualize drug location on the droplet surface
In some ranges of PFC/copolymer concentration ratios, micelles coexist with nanodroplets (zone 2, Fig. 13.2). As PFC concentration increases, all block copolymer is used for droplet stabilization and micelles disappear; only droplets are observed in zone 3. The droplet size in PFC nanoemulsions ranges from 200 to 750 nm depending on the type of the stabilizing copolymer, perfluorocarbon-to-copolymer concentration ratio and emulsification conditions (Gao et al. 2008). An example of a size distribution diagram for a 1 %PFCE/5 %PEG-PDLA formulation is presented in Fig. 13.4, revealing coexisting micelles and nanodroplets (corresponding to zone 2 of the phase diagram).
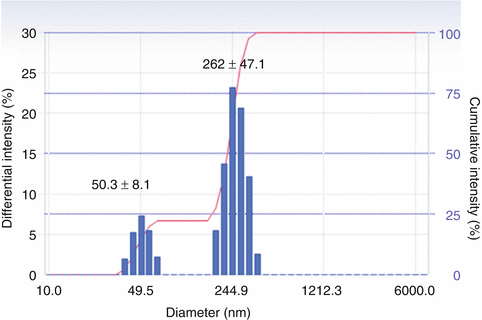
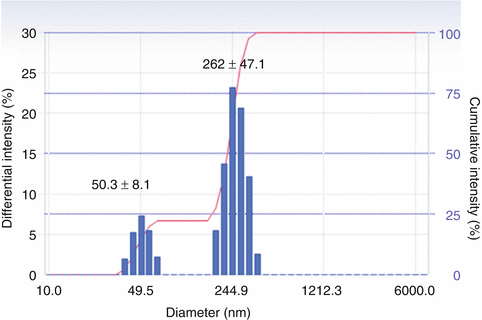
Fig. 13.4
Particle size distribution in a 1 % PECE/5 % PEG-PDLA formulation (zone 2 of the phase diagram, see Fig. 14.2); left peak – PEG-PDLA micelles with dissolved PFP; right peak – PFCE nanodroplets. Only one (nanodroplets) peak would be observed in zone 3 (i.e. at higher PFP concentrations). Particle sizes in all zones depend on PFCE and PEG-PDLA concentrations and concentration ratios (Reprinted with permission from (Rapoport et al. 2013))
13.3.2 Droplet-to-bubble Phase Transition in PFP Nanoemulsions
Starting with a pioneering work by Apfel, it was shown that perfluorocarbon droplets can convert into microbubbles under ultrasound irradiation (Apfel 1998). This effect, called acoustic droplet vaporization (ADV), has been thoroughly investigated by the Fowlkes group (Fabiilli et al. 2009, 2010a, b; Kripfgans et al. 2000, 2002, 2004, 2005; Lo et al. 2006, 2007; Miller et al. 2000; Wong et al. 2011; Zhang et al. 2010), the De Jong and Versluis groups (Chen et al. 2013; Faez et al. 2013; Kokhuis et al. 2013; Kooiman et al. 2014; Maresca et al. 2013; Reznik et al. 2013, 2014; Segers and Versluis 2014; Shpak et al. 2013, 2014; Ten Kate et al. 2013; Thomas et al. 2013) and Rapoport group (Gao et al. 2008; Nam et al. 2009; O’Neill and Rapoport 2011; Rapoport et al. 2009a, b, 2010a, 2012a). In medical applications, acoustic droplet vaporization was tested for temporal and spatial control of tissue occlusion (Kripfgans et al. 2002; Kripfgans et al. 2005; Zhang et al. 2010), as well as for cavitation nucleation agents for non-thermal ultrasound therapy (Miller et al. 2000; Miller and Song 2002), for enhancing gene transfer, for phase aberration correction (Kripfgans et al. 2002) and for the ultrasound-enhanced drug delivery; for reviews on the latter application, see (Rapoport 2012a, b).
13.3.2.1 Vaporization of the PFP Droplets: Mechanism and Therapeutic Applications
It was found that micrometer-sized albumin-coated PFP droplets vaporized into gas bubbles with the application of short tone bursts in the diagnostic frequency range of 1.5–8 MHz (Kripfgans et al. 2000). The resulting bubbles were 20–80 μm in diameter. The threshold for vaporization decreased with increasing ultrasound frequency and sonication time, and in the presence of microbubbles as nucleation agents (Kripfgans et al. 2000; Lo et al. 2007). The vaporization threshold was higher for smaller droplets (Kripfgans et al. 2004). These experiments were complemented with the optical imaging of the droplet-to-bubble transition using the ultra-high speed imaging camera (Wong et al. 2011).
Physico-chemical aspects of the PFP droplet vaporization were discussed by Rapoport et al. (2009a, b, 2010a). PFP has a boiling temperature of 29 °C at atmospheric pressure and therefore manifests high propensity for vaporization under heating. However, for small droplets stabilized by elastic copolymer shells, the Laplace pressure (i.e. the pressure difference between the inside and outside of a droplet) may substantially increase boiling temperature. This effect is caused by the surface tension at the interface between a droplet and a bulk liquid. The Laplace pressure is given by
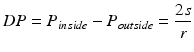
where P inside is the pressure inside a droplet, P outside is the pressure outside a droplet, σ is the surface tension and r is a droplet radius.
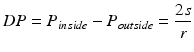
(13.1)
Excessive pressure inside a droplet results in an increase in PFP boiling temperature. This phenomenon has important consequences for drug delivery. Laplace pressure is inversely proportional to droplet size according to Eq. 13.1, meaning smaller droplets have higher boiling temperatures than larger droplets. The surface tension at the PFP/water interface for “naked” (i.e. not surfactant-coated) PFP droplets is 56 ± 1 mN/m. Using the known parameters of the Antoine equation for the pressure dependence of PFP vaporization temperature (Barber and Cady 1956), the dependence of the PFP droplet vaporization temperature on droplet size was calculated for two values of the interfacial tension 30 and 50 mN/m, typical values for PEG-coated colloid particles (Alexandridis et al. 1994) (Fig. 13.5) (Rapoport et al. 2009a). As indicated by Fig. 13.5, PFP boiling temperature in droplets smaller than 4 μm is higher than physiological temperature. These droplets remain in the liquid state at physiological temperatures, while droplets larger than 4 μm vaporize. Therefore, droplets larger than 4 μm should be excluded from pharmaceutical PFP emulsion formulations as they would prematurely vaporize upon systemic injection. The fact that micron-sized droplets would not extravasate is equally important. Hence, in Rapoport’s works, paclitaxel (PTX)-loaded PFP nanodroplets were prepared with the average size of 250–300 nm and relatively low polydispersity (see Fig. 13.4). These nanodroplets circulated as liquid nanodroplets and gradually extravasated into tumor tissue, upon which their conversion into microbubbles, which led to a localized drug release, was triggered by tumor-directed ultrasound.
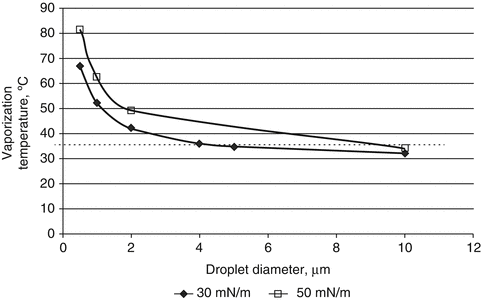
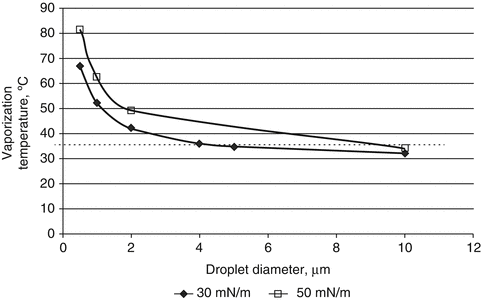
Fig. 13.5
Dependence of the PFP boiling temperature in nanodroplets on droplet size calculated for two characteristic values of interfacial energy, 30 and 50 mN/m (Reprinted with permission from (Rapoport et al. 2013))
Note of warning: Droplet-to-bubble transition upon injection of PFP nanoemulsion may be induced by shear stresses generated during injection through a small diameter (high-gauge) needle (Rapoport et al. 2009a, b). This phenomenon was first observed in Echogen microemulsions; its clinical implications have been discussed in ref. (Becher and Burns 2000). Though some generation of microbubbles in the vascular bed may be beneficial for increasing ultrasound-induced vascular permeability (Caskey et al. 2007, 2009a, b; Chen et al. 2010; Chen et al. 2011; Kinoshita et al. 2006; Matula and Guan 2011; Stieger et al. 2007), substantial transition of nanodroplets into microbubbles inside blood vessels should be avoided unless occlusion therapies have been considered. For safety reasons, PFP nanoemulsions should be injected either by infusion or through low-gauge needles.
Upon complete PFP droplet vaporization inside a copolymer wall, particle diameter increases fivefold due to a 125-fold density difference between liquid and gaseous PFP phases (Gao et al. 2008; Kripfgans et al. 2000; Rapoport et al. 2007). Therefore, a 500-nm diameter droplet would generate a 2.5-μm bubble upon complete vaporization. However, bubbles of much larger sizes were observed upon ultrasound-induced PFP droplet vaporization (Kripfgans et al. 2000; Lo et al. 2007; Rapoport et al. 2009a, b). These were secondary bubbles formed from primary 2.5-μm bubbles. At least two different mechanisms of bubble growth beyond the size of primary bubbles may be considered: bubble coalescence with droplets or between themselves; diffusion of dissolved air and/or PFP from small bubbles into larger bubbles (i.e. Oswald ripening). Oswald ripening may also play a predominant role in gel or solid matrices, where droplet and bubble diffusion and collisions are restricted or stalled.
The increase of bubble size upon ultrasound-induced vaporization was tested in embolotherapy. Experiments performed on the externalized rabbit kidney using albumin-coated PFP microdroplets with an initial diameter less than 6 μm showed more than a 70 % perfusion reduction following ADV (Kripfgans et al. 2005). The authors hypothesized that this effect may be sufficient for cell death and tumor treatment via ischemic necrosis. It was also suggested that radiofrequency ablation of tumors might also benefit from ADV due to reduced perfusion and heat loss. These experiments were later extended to externalized canine kidneys (Zhang et al. 2010). Substantial reduction of cortex perfusion was achieved in some cases.
To elucidate physical mechanisms behind acoustic vaporization of PFP droplets, the relationship between ADV and inertial cavitation (IC) thresholds was studied (Fabiilli et al. 2009; Giesecke and Hynynen 2003; Kawabata et al. 2006; Lo et al. 2007; Schad and Hynynen 2010). Most of these experiments were performed with albumin- or lipid-coated microbubbles and showed that the ADV threshold was lower than the inertial cavitation threshold, indicating that the droplet-to-bubble transition preceded inertial cavitation. Measurements of the inertial cavitation threshold were performed for micrometer-sized albumin-shelled droplets containing various PFC cores, including those with higher boiling temperatures than that of PFP (i.e. perfluorohexane and perfluoromethylcyclohexane) (Giesecke and Hynynen 2003). The authors found that inertial cavitation thresholds did not noticeably depend on perfluorocarbon molecular weights and boiling temperatures, and thus, the droplets did not need to be in a superheated state to be cavitated by ultrasound bursts. This was later confirmed in experiments with nanosized perfluoro-15-crown-5-ether (PFCE) droplets that effectively converted into bubbles at ultrasound pressures that were only slightly higher than those for PFP nanodroplets (Rapoport et al. 2011). The mechanism of a droplet-to-bubble conversion with high boiling temperature perfluorocarbon compounds is most probably fundamentally different from true vaporization (see below). It was also found that nanometer-sized droplets containing a mixture of perfluoropentane (DDFP) and 2H,3H-perfluoropentane could be vaporized at diagnostic ultrasound frequencies (4–7.8 MHz) and that the vaporization threshold could be changed by altering relative concentrations of the two PFCs in the droplet (Kawabata et al. 2005). The authors hypothesized that the vaporization of a higher boiling temperature 2H,3H-perfluoropentane may have been caused not only by a directly delivered ultrasound energy, but also by the energy deposited by ultrasonically induced PFP bubbles. Droplet-to-bubble transition in PFP nanodroplets was shown to be catalyzed by pre-existing larger droplets or microbubbles. The catalytic effect was stronger at lower ultrasound frequencies (Rapoport et al. 2009a, b). This suggested that the droplet-to-bubble transition in nanoscaled droplets can be effectively catalyzed not only by mixing PFCs of various boiling temperatures, but also by using a bimodal or broad droplet size distribution in initial formulations. This is given that the conversion of larger droplets at lower power levels would catalyze droplet-to-bubble transition of smaller droplets.
Given that there is a large mismatch between the ultrasound wavelength and the droplet size, detailed physical mechanisms of droplet vaporization today remain elusive. Nevertheless, a new mechanism was recently proposed (Kooiman et al. 2014; Reznik et al. 2014; Shpak et al. 2014). The authors concluded that droplet vaporization is initiated by a combination of two phenomena: highly nonlinear distortion of the acoustic wave before it hits the droplet, and focusing of the distorted wave by the droplet itself. At high excitation pressures, nonlinear distortion causes significant superharmonics with wavelengths of the order of the droplet size. These superharmonics strongly contribute to the focusing effect; therefore, this proposed mechanism also explains the observed pressure thresholding effect. This interpretation is validated with experimental data on the positions of the nucleation spots captured with an ultrahigh-speed camera; excellent agreement with the theoretical predictions was observed. The authors suggested a number of ways to decrease ultrasound pressure required for droplet vaporization: mixing different liquids to vary the acoustic impedance; to use dual or multiple frequency transducers to optimize the amplitudes and phases of the transmitted waves for maximal constructive interference, thus maximizing the focusing on the droplet. Still, the conclusions of the paper were not promising for delivering nanodroplet-loaded drugs into tumor interstitium using the ADV effect because nucleation of vaporization strongly depended on droplet sizes and manifested low probability for nanosized droplets. Small droplets would require higher ultrasound frequencies for vaporization; the latter don’t penetrate deep into the interior of the body due to enhanced attenuation. On the other hand, micron-sized droplets that require lower ultrasound frequencies for nucleation and manifest higher probability of vaporization would not extravasate. Despite these apparent issues, excellent therapeutic results were obtained with drug-loaded nanosized droplets and ultrasound (see Sect. 13.4). Note that droplet-to-bubble transition is not necessarily associated with true perfluorocarbon vaporization as discussed in the next section.
13.3.2.2 Droplet-to-bubble Phase Transitions in Perfluoro-15-crown-5-ether Nanoemulsions
PFCE has a much higher boiling temperature than PFP (146 °C at atmospheric pressure). However, initiating droplet-to-bubble transition in PFCE nanodroplets required only slightly higher ultrasound energies than those for PFP (Rapoport et al. 2011), confirming the previously reported data (Giesecke and Hynynen 2003). The droplet-to-bubble transition in PFCE nanodroplets was induced by both continuous wave or pulsed ultrasound; the latter caused insignificant heating (Rapoport et al. 2011), confirming that the mechanism of droplet-to-bubble transition in a PFCE nanoemulsion was non-thermal. A possible mechanism of ultrasound-induced droplet-to-bubble transition in PFCE has been suggested (Rapoport et al. 2011). Perfluorocarbon emulsions differ from other emulsions by very high gas solubility, particularly for oxygen. This feature has allowed the use of perfluorocarbon emulsions as blood substitutes (Cohn and Cushing 2009). According to Henry’s law, the solubility of gases increases with pressure. It has been hypothesized that a pressure drop during a rarefactional phase of ultrasound led to the evolution of PFC-dissolved oxygen in a gas phase inside the nanodroplet shell, followed by a rectified diffusion of dissolved gases from the surrounding liquid into the resulting nanobubble. According to this hypothesis, PFCE bubbles should predominantly contain a mixture of oxygen and other ambient gases, rather than PFCE vapor (though a bubble will also contain a low fraction of PFCE vapor in equilibrium with PFCE liquid phase). The bubbles formed this way are transient in nature; when the ultrasound is turned off, the equilibrium between nanodroplets and surrounding medium is restored; gases with super-equilibrium concentrations diffuse out of bubbles, thus restoring PFCE nanodroplets. This was confirmed experimentally. A PFCE nanoemulsion was placed in a long test tube; only the lower part of a test tube was sonicated. Bubbles that formed in the ultrasound field moved up due to buoyancy, but converted back to heavy droplets upon leaving the ultrasound field. On the way down towards the bottom of the test tube, droplets entered the ultrasound field and converted back to bubbles. Ultimately, nanoparticles “danced” at the border of the ultrasound field.
This proposed mechanism has been corroborated by the fact that degassing PFCE nanoemulsions inhibited the droplet-to-bubble transition that was restored after contact with air was re-established. The bubble formation mechanism suggested above is different from true vaporization of nanodroplets, which is involved in the formation of PFP bubbles. Bubbles formed from either PFP or PFCE nanodroplets were shown to cavitate in the ultrasound field, as manifested by the generation of harmonic frequencies in the fast Fourier transform (FFT) spectra of the scattered ultrasound beam (Rapoport et al. 2009a, 2010a, 2011). Stable cavitation was observed in both an aqueous environment and agarose gel used as a tissue phantom (Fig. 13.6a). However, a broadband noise (characteristic of inertial cavitation) was not generated by PFCE droplets (Fig. 13.6b). Droplet-to-bubble conversion in-vivo was recently confirmed by ultrasound imaging (Matsunaga et al. 2012; Wilson et al. 2012). The material presented above implies that drug-loaded nanodroplets may serve as nano-scaled microbubble precursors that have a prospect of accumulating in tumors due to their nanoscaled sizes, and then convert into microbubbles in-situ under the action of tumor-directed ultrasound. Using this approach, successful therapy for breast, ovarian, and pancreatic cancer was achieved in animal model preclinical studies as described below.
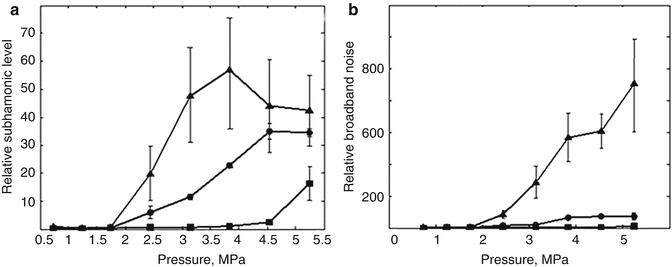
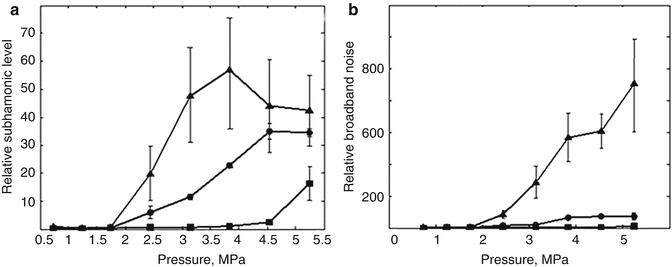
Fig. 13.6
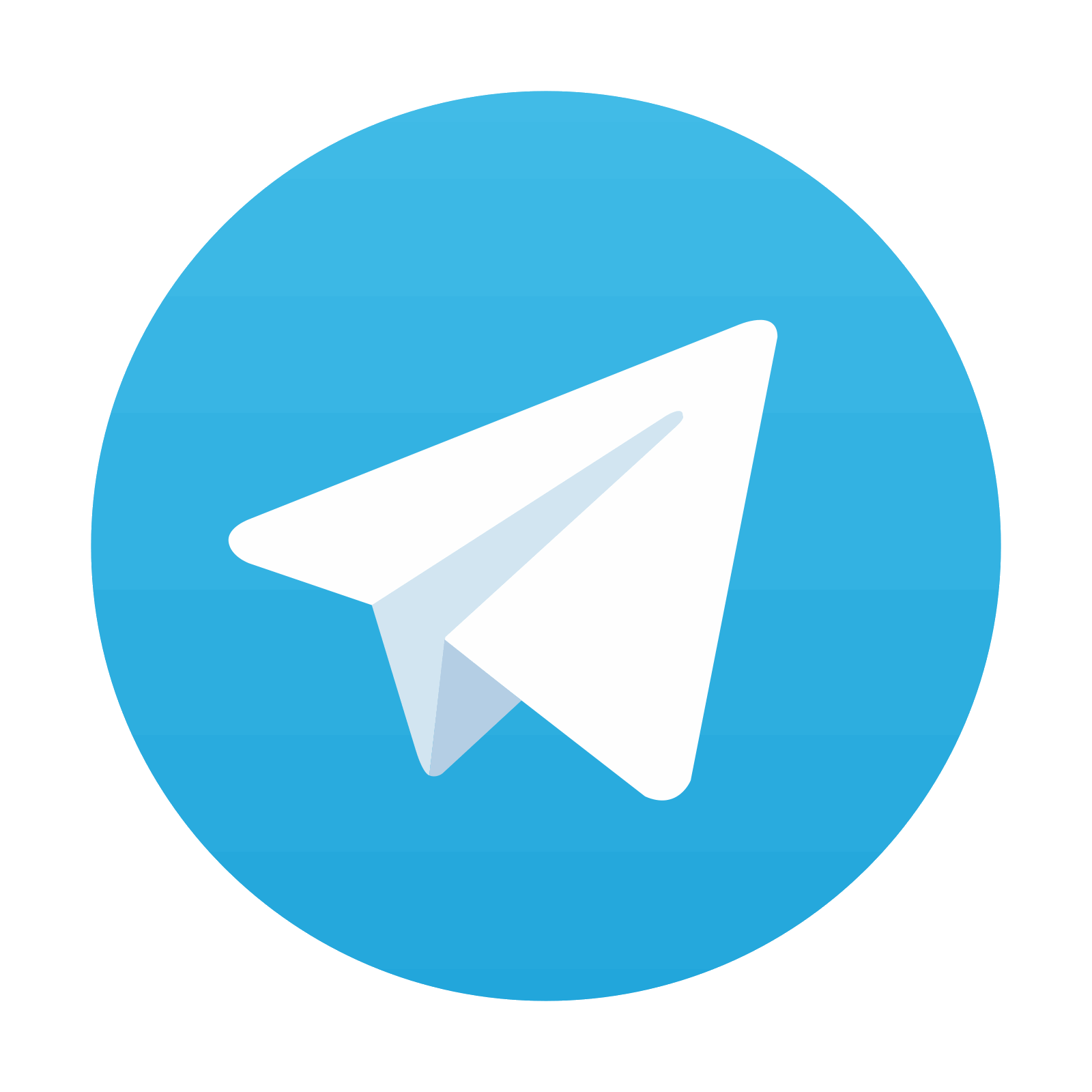
Relative subharmonic (a) and broadband noise (b) levels generated under the action of 1-MHz focused ultrasound (HIFU) at room temperature by PFP and PFCE nanodroplets inserted into 0.6 % agarose gel. Nanodroplets were stabilized by a mixture of 0.25 % PEG-PLAA/0.25 % PEG-PCL copolymer. Triangles – PFP droplets; circles – PFCE droplets; squares – pure agarose gel (Reprinted with permission from (Rapoport et al. 2010a))
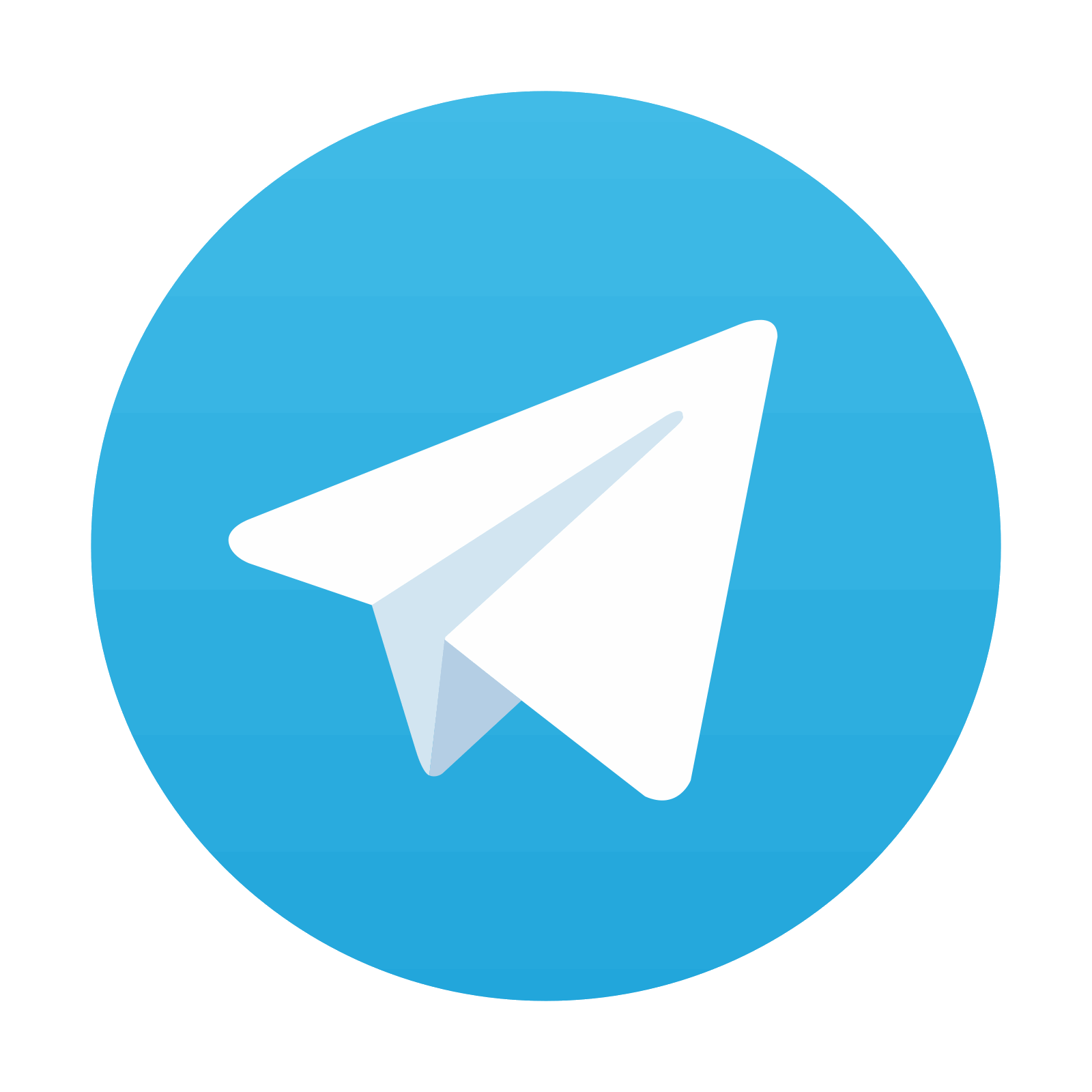
Stay updated, free articles. Join our Telegram channel
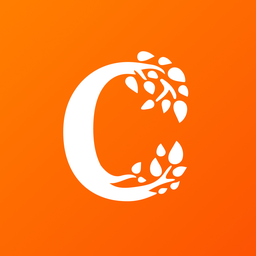
Full access? Get Clinical Tree
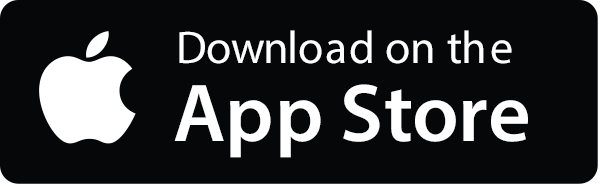
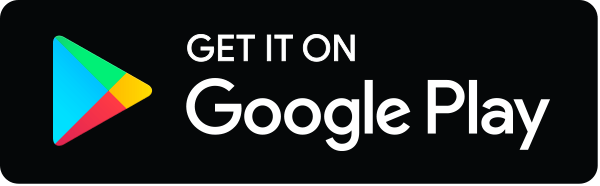
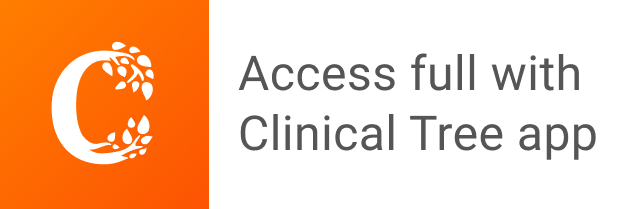