Evaluation of Myocardial Perfusion
Keiichiro Yoshinaga
Nagara Tamaki
Terrence D. Ruddy
Robert A. deKemp
Rob S. B. Beanlands
Coronary artery disease (CAD) continues to be a major cause of death not only in modern industrialized society but also in developing countries. Techniques for evaluating myocardial blood flow (MBF) plays an important role in the identification of patients with CAD and determination of their prognosis. Patients at high risk for subsequent cardiac events can be treated with aggressive interventional therapy and low-risk patients managed medically. Measurement of regional myocardial perfusion permits measurement of coronary flow reserve and the evaluation of the physiologic significance of coronary lesions and adequacy of collateral supply. These MBF measurements are often essential for characterizing the functional significance of CAD and are complementary to coronary angiography. As well, positron emission tomography (PET) measurements of MBF reserve and endothelial function are useful for the serial evaluation of patients with the goals of determining the response to therapy and progression of disease. In the past the clinical use of cardiac PET has been limited by the cost of the technology, but the development of less expensive PET cameras and the increased use of PET for other indications have resulted in wider clinical application of cardiac PET as well. Furthermore, PET is uniquely suited to be a quantitative research tool for studies evaluating myocardial perfusion in vivo in man.
PET has become accepted as the most accurate technique for the measurement of regional MBF. Tissue perfusion in milliliters per minute per gram of weight can be measured in vivo by relating myocardial tracer kinetics to arterial tracer input. The accuracy of these noninvasive measurements requires (a) a radiotracer with retention or clearance kinetics related to MBF during normal and pathophysiologic states, (b) accurate measurement of the arterial blood and myocardial activity of the radiotracer with adequate temporal resolution to define the tracer kinetics, and (c) established
methods of modeling of the tracer kinetics to permit calculation of regional flow measurements. Current PET technology has spatial resolution of 4 to 6 mm and accurate attenuation correction, permitting measurement of myocardial radiotracer concentration at frequent time intervals. Integration of PET instrumentation with a computed tomography (CT) scanner (PET/CT) can provide the means for accurate attenuation correction of myocardial perfusion imaging (MPI) and coronary arteries anatomical information in a single setting. Suitable kinetic modeling has been developed for several PET flow tracers and makes the accurate measurement of tissue blood flow possible (1,2,3,4,5,6,7).
methods of modeling of the tracer kinetics to permit calculation of regional flow measurements. Current PET technology has spatial resolution of 4 to 6 mm and accurate attenuation correction, permitting measurement of myocardial radiotracer concentration at frequent time intervals. Integration of PET instrumentation with a computed tomography (CT) scanner (PET/CT) can provide the means for accurate attenuation correction of myocardial perfusion imaging (MPI) and coronary arteries anatomical information in a single setting. Suitable kinetic modeling has been developed for several PET flow tracers and makes the accurate measurement of tissue blood flow possible (1,2,3,4,5,6,7).
Table 11.1.1 PET Myocardial Blood Flow Tracers | ||||||||||||||||||||||||||||||||||||||||
---|---|---|---|---|---|---|---|---|---|---|---|---|---|---|---|---|---|---|---|---|---|---|---|---|---|---|---|---|---|---|---|---|---|---|---|---|---|---|---|---|
|
Myocardial Blood Flow Tracers
The commonly used PET blood flow tracers can be divided into (a) inert freely diffusible tracers such as oxygen-15 ([15O])-labeled water and (b) physiologically retained tracers such as nitrogen-13 ([13N])-ammonia and rubidium-82 ([82Rb]). The commonly used PET MBF tracers are listed in Table 11.1.1 in order of increasing half-life.
Diffusible tracers such as [15O]-labeled water diffuse freely across membranes, resulting in a distribution of tracer between vascular and extravascular space related to the partition coefficient. This partition coefficient for [15O]-labeled water is stable over a wide range of flow rates such that uptake of [15O]-labeled water is not diffusion limited. Conversely, the extraction fraction of physiologically retained radiotracers decreases with increasing blood flow and results in underestimation of MBF based on the measurement of tissue uptake. These physiologic properties are incorporated into the kinetic models for determination of MBF.
Nitrogen-13-Ammonia
[13N]-ammonia is the most commonly used myocardial perfusion tracer in PET centers with an on-site cyclotron. Nitrogen-13 is produced with a cyclotron and a water target via the [16O] (p,α) [13N] nuclear reaction to yield the radionuclide. Ammonium ions can be converted readily with reducing agents.
Following intravenous administration of [13N]-ammonia, [13N]-ammonia crosses capillary and cell membranes via passive diffusion and is retained in myocardial tissue by the incorporation of the label into the amino acid pool as glutamine. The synthesis of glutamine is the rate-limiting process of tissue retention of [13N]-ammonia. Nitrogen-13-ammonia in blood is in an equilibrium state with ionic [13N]-ammonium (8,9). The first pass extraction fraction is nearly 100%, since [13N]-ammonia diffuses freely across membranes (9). In myocardium, [13N]-ammonia is either incorporated into synthesis of [13N]-glutamine or back-diffuses into the vascular space (10). The net extraction fraction is approximately 80% in the resting flow range, but decreases with higher flow rates.
The image quality achieved with [13N]-ammonia is considered to be the best among all of the PET perfusion tracers due to the relatively long physical half-life, relatively high extraction fraction, low background, and low positron energy (Fig. 11.1.1).
Rubidium-82
[82Rb] is produced from a strontium-82/rubidium-82 generator that can be eluted every 10 minutes (11). [82Rb] is a widely used PET perfusion tracer in centers without immediate access to an on-site cyclotron (7,12). The half-life of the parent isotope is 25.5 days and results in a generator life of about 4 to 6 weeks. Not needing a cyclotron is a major advantage of this radiotracer. Although the commercial generator is expensive, a high volume of cardiac studies significantly reduces the cost of radiotracer per examination. The short physical half-life of 76 seconds makes [82Rb] suitable for repeated and sequential perfusion studies (Fig. 11.1.2). As well, the short half-life necessitates rapid image acquisition shortly after tracer administration. The relatively high positron energy of [82Rb] of 3.15 MeV is associated with a positron range averaging 7.5 mm and results in lower spatial resolution than seen with [13N]-ammonia with a positron range of 2.5 mm.
[82Rb] is an analogue of potassium and has similar biological activity to thallium-201 [201Tl] (13,14). [82Rb] is rapidly extracted from the blood and concentrated by the myocardium. In animal models, the first-pass extraction fraction is 50% to 60% at rest and decreases to 25% to 30% at peak flow (13,15,16). In addition, the extraction fraction may remain reduced in myocardium recovering from transient ischemia (17). This radiotracer is retained in the myocardium and equilibrates in the potassium pool. Thus, cell membrane disruption may cause rapid tissue loss of radioactivity, and [82Rb] kinetics can be used as a marker of tissue viability (18).
A small and mobile generator infusion system is used for eluting [82Rb] every 10 to 15 minutes with low radiation exposure to personnel or patient (11). Quantitative assessment of MBF and flow reserve is quite feasible and clinically practical with this generator as compared to cyclotron-produced compounds (19,20,21,22,23).
Copper-62 PTSM
Copper-62 ([62Cu]) pyruvaldehyde bis (N4-methylthiosemicarbazone) (PTSM) is another generator-produced PET perfusion tracer and is produced from zinc-62 ([62Zn])/copper-62 generator (24,25). [62Cu] PTSM is quite suitable for serial measurement of MBF since the physical half-life of 9.7 minutes is short (26). Unfortunately, the relatively short half-life of 9.2 hours of the parent, [62Zn], results in the need for a fresh generator on a daily basis.
Following intravenous administration of [62Cu] PTSM, the radiotracer clears rapidly from blood with high tracer uptake in the myocardium. The uncharged lipophilic copper PTSM rapidly diffuses across cell membranes. Within the cell, the [62Cu] PTSM is
susceptible to reductive decomposition by reaction with ubiquitous intracellular enzymes. As a result, an effectively irreversible deposition of ionic copper is seen in the cells.
susceptible to reductive decomposition by reaction with ubiquitous intracellular enzymes. As a result, an effectively irreversible deposition of ionic copper is seen in the cells.
[62Cu] PTSM is a promising tracer for the evaluation of myocardial and cerebral perfusion. High-quality myocardial perfusion images can be obtained shortly after tracer administration in the animal as well as human studies (26,27). Five percent to 10% of the injected dose of copper PTSM remains in the circulation due to binding to red blood cells. Therefore, the quantitative measurement of regional MBF using the microsphere model requires correction of the arterial blood time activity curve for blood pool binding (28). A significant reduction of extraction fraction is observed in the high flow range and requires correction to express data as absolute MBF (28).
Oxygen-15-Water
[15O]-labeled water was one of the first radiopharmaceuticals developed for PET use and is considered the gold standard for quantification of myocardial perfusion. [15O]-labeled water is usually obtained from [15O]-oxygen gas combined with hydrogen gas. The [15O] gas is produced either by [14N] (d,n) [15O] reaction or [15N] (p,n) [15O] method. Since the physical half-life of [15O] is only 2 minutes, an in-house cyclotron is required to use [15O]-labeled water.
One of the major advantages of [15O]-labeled water is feasibility for quantitative assessment of MBF.
Carbon-11-Butanol
Radiolabeled aliphatic alcohols have been evaluated in the search for better blood flow tracers. Butanol seems to be a nearly optimal flow tracer since it has a better partition coefficient than [15O]-labeled water. Carbon-11 ([11C])-butanol can be produced with a simple synthesis with a high yield (30,31). However, this tracer has been mainly used for cerebral perfusion studies and not yet well evaluated for measurement of MBF.
Fluorine-18 Fluorobenzyl Triphenyl Phosphonium
Fluorine-18 ([18F])-labeled compounds have relatively long physical half-life (110 minutes) and can be delivered to PET centers without an on-cite cyclotron in the same manner as [18F]-fluorodeoxyglucose (FDG). Among [18F]-labeled radio tracers, [18F]-fluorobenzyl triphenyl phosphonium ([18F]-FBnTP) represents a promising tracer. [18F]-FBnTP is rapidly accumulated to myocardium and has a long retention time. Thus, [18F]-FBnTP yielded high quality images in preliminary canine studies (32). This early results are encouraging (Fig. 11.1.2A). In healthy dogs, FBnTP accumulates rapidly in the myocardium (time to plateau <60 seconds) and distributes uniformly throughout the myocardium (6% to 8% coefficient of variance). In dogs with coronary stenosis, FBnTP flow defect contrast was 2.7 times greater than tetrofosmin ex vivo. A near identical qualitative and quantitative estimate of stenosis severity was obtained by early, short (5 to 15 minutes) and delayed, prolonged (30 to 60 minutes) [18F]-FBnTP PET scans. Although animal results are promising, this tracer needs further investigation in human studies. An additional PET tracer, labeled with [18F], has recently been reported, RP-1012-18. This agent is a structural analogue of pyridaben, a known mitochondrial complex 1 (MC-1) inhibitor (Figs. 11.1.2B, 11.1.2C).
Clinical Indication and Imaging Protocols
Clinical Indication
Current clinical guidelines (33) and a joint position statement (34) address patients with intermediate likelihood of CAD when the patient has had a nondiagnostic or equivocal single-photon emission computed tomography (SPECT) myocardial perfusion imaging (MPI) or other noninvasive imaging tests. For such patients, indications for PET MPI for diagnosis and detection of ischemia are considered class I (level of evidence B). In general, patients who are unable to exercise or have left bundle branch block (LBBB) or ventricular pacing rhythm may also benefit from PET MPI for diagnosis and detection of ischemia (class IIa; Class I [level of evidence B]) (Fig. 11.1.3) (33,34).
Imaging Protocol
Patient Preparation
Patients should be instructed to fast for 6 hours or more, to abstain from caffeine-containing products (i.e., coffee, tea, chocolate, and cola) at least 12 hours, and to avoid theophylline-containing medications for 48 hours prior to adenosine/adenosine triphosphate
(ATP)/dipyridamole pharmacological stress tests (35). Patients who would have dobutamine stress tests should stop β-blockers 48 hours before the tests if the goal is diagnosis of CAD and if it is clinically safe to do so.
(ATP)/dipyridamole pharmacological stress tests (35). Patients who would have dobutamine stress tests should stop β-blockers 48 hours before the tests if the goal is diagnosis of CAD and if it is clinically safe to do so.
Stress Protocol
Vasodilator Pharmacological Stress
Adenosine, ATP, and dipyridamole stress are the established clinical stress methods for myocardial perfusion PET imaging. These vasodilator agents block transport of adenosine into the cells and/or increase extra cellular levels of adenosine, which causes coronary vasodilatation by interacting with the adenosine A2 receptors in the cell membrane. Activation of A2 receptors dilates coronary vasculatures via the production of adenylate cyclase and cyclic adenosine monophosphate stimulation of potassium channels, which results decreased intracellular calcium uptake. These agents increase MBF and permit measurement of MBF reserve. Adenosine and dipyridamole increase MBF by three- to fivefold in normal coronary territories without increasing oxygen demand. In contrast, myocardial regions supplied by diseased coronary arteries already have dilated coronary arterioles at rest in order to maintain resting blood supply. Thus, the hyperemic response is attenuated and coronary flow reserve is reduced (5).
Dipyridamole (0.56 mg/kg) is infused intravenously over 4 minutes. The radiotracer should be injected 3 to 5 minutes after the completion of dipyridamole infusion (Fig. 11.1.4). Adenosine should be administered as a continuous infusion (140 μg/kg/min) over a 6-minute period, and the radiotracer should be injected at the 3-minute mark of the 6-minute infusion period (36).
Dobutamine Stress
Dobutamine stress is a feasible alternative in the situation when adenosine, ATP, or dipyridamole is contraindicated because of severe reactive airway disease or caffeine intake (36). However, dobutamine perfusion imaging has not been as extensively studied as vasodilator stress agents. Dobutamine increases regional MBF based on physiologic principles of coronary flow reserve. Flow increases to meet increasing demand. However, this response is attenuated during high-dose dobutamine administration (20 to 40 μg/kg/min) in segments supplied by diseased vessels.
In practice, dobutamine can start 5 to 10 μg/kg/min, and dobutamine dose can be increased 5 μg/kg/min at 3-minute intervals up to a maximum dose of 40 μg/kg/min to achieve >85% of target heart rate (0.85 × [220 – age]).
Exercise Stress
An exercise stress test can evaluate the imbalance of oxygen demand and blood supply and thus provides physiological information as well as functional capacity. Although exercise stress is considered to be a physiological stress and is widely applied for SPECT myocardial perfusion imaging, in general pharmacological stress is preferred in PET imaging because imaging starts shortly after tracer administration and pharmacological stress can better avoid body movements during image acquisitions. However, [13N]-ammonia can be used in conjunction with treadmill or upright bicycle exercise test. The tracer is administrated at peak exercise and exercise should be continued for an additional 30 to 60 seconds after tracer injection. The patient is then repositioned in the PET camera to start the acquisition within 4 to 6 minutes (37,38,39). Accurate repositioning is important to minimize artifact due to incorrect attenuation correction. A bicycle ergometer can be attached to the PET bed, which makes this approach more feasible in terms of attenuation correction (39), although patient body position may shift during the supine exercise. Exercise stress is also available for the [82Rb] imaging (35,40). Using treadmill exercise, patients should continue their peak exercise 1.0 to 1.5 minutes after [82Rb] intravenous
administration. The patient is then repositioned in the PET scanner and the image acquisition should begin approximately 3 minutes after tracer injection (35,40).
administration. The patient is then repositioned in the PET scanner and the image acquisition should begin approximately 3 minutes after tracer injection (35,40).
Image Acquisition and Processing
Nitrogen-13-Ammonia
For relative perfusion imaging, [13N]-ammonia is injected as a bolus of 10 to 20 mCi (370 to 740 MBq) and static images are acquired 1.5 to 3 minutes after tracer administration for an imaging time of 5 to 15 minutes (35).
For quantitative measurement of MBF, serial dynamic PET imaging begins simultaneously with tracer administration. From the dynamic images, time activity curves are generated for the myocardium and the blood pool. Global and regional MBF can be measured with use of compartmental tracer kinetic modeling fit to myocardial activity data and corrected for the arterial input function. Previously, the arterial input function was measured by sequential arterial blood sampling. However, blood pool time activity data obtained from left ventricular cavity provides an accurate measurement without the use of arterial cannulation and makes acquisitions more feasible and easier for the patient.
Rubidium-82
A large amount of tracer can be administered to the patient because of the short physical half-life of [82Rb]. Forty to 60 mCi (1,480 to 2,220 MBq) of [82Rb] can be injected as a bolus of 30 seconds or less followed by serial dynamic acquisition using a PET camera with a high-count rate and high sensitivity with a short acquisition time (35). After a 70- to 150-second completion of tracer injection, a 3- to 7.5-minute image acquisition is initiated (35,41). Alternatively, the newer generation of PET cameras can acquire data in three-dimensional modes and without septa, resulting in higher sensitivity and the need for smaller doses of [82Rb] (19).
For quantitative assessment of regional MBF, a two-compartment model has been used, which includes activity in the vascular space and within the tissue compartment (42). Following bolus injection of the tracer, predominantly unidirectional transport is assumed from the vascular space into the tissue space. In the canine model, regional MBF can be accurately estimated using [82Rb] (43). A simplified approach using a summed late image corrected for the input function, similar to that described for [13N]-ammonia, has also been used. This approach allows reasonable quantification for perfusion that may be easier to apply in the clinical setting (Fig. 11.1.5) (19,21,22).
Semiquantitative Image Interpretation
Myocardial perfusion defects are usually identified by visual analysis of the reconstructed slices. Such perfusion defects should be characterized by their location as they relate to a specific coronary artery, their extent, and the defect severity. Defects noted during stress imaging whose severity and extent improve at rest are typically categorized as reversible, which indicates myocardial ischemia (Fig. 11.1.6). On the other hand, defects that are fixed in extent and severity at both stress and rest are categorized as myocardial injury or infarction. Perfusion defect description, including extent, severity, reversibility, location, and specific coronary territories, should be reported routinely (44). Moreover, cardiac event risk over 2 to 3 years may be addressed in the report based on the following risk stratifications guidelines that recommend semiquantitative analysis: normal perfusion = 0, mild = 1, moderate = 2, severe = 3, and absent uptake = 4, using a 17-segment model (Figs. 11.1.7A, 11.1.B) (34,45). Using this approach, the summed perfusion defect score can be calculated and is useful for cardiac risk stratification (34,46). A number of previous SPECT data have confirmed the critical cutoff for abnormal scan is summed stress score >4 in a 20-segment model (34). Berman et al. (47) confirmed this cutoff value can apply for the currently recommended 17-segment model in SPECT perfusion studies. Yoshinaga et al. (41) also reported that abnormal cutoff value of SSS 4 or greater can apply to [82Rb] PET studies with the 17-segment model.
Common Artifacts with Nitrogen-13-Ammonia and Rubidium-82
[13N]-ammonia studies in normal volunteers have shown a slight heterogeneity of regional tracer retention (Fig. 1.11.1) (29). [13N]-ammonia retention was decreased by 10% in the lateral wall of the left ventricle as compared to the septum. The underlying mechanism of
this phenomenon is poorly understood. However, this mild heterogeneity has to be appreciated when interpreting [13N]-ammonia perfusion images. Changes in the metabolic and hemodynamic environment within physiologic ranges do not significantly alter the retention of [13N]-ammonia (9,10). Other factors to consider with [13N]-ammonia imaging include excess liver activity, which can interfere with evaluation of the inferior wall, and excess lung activity in patients with pulmonary disease or congestion.
this phenomenon is poorly understood. However, this mild heterogeneity has to be appreciated when interpreting [13N]-ammonia perfusion images. Changes in the metabolic and hemodynamic environment within physiologic ranges do not significantly alter the retention of [13N]-ammonia (9,10). Other factors to consider with [13N]-ammonia imaging include excess liver activity, which can interfere with evaluation of the inferior wall, and excess lung activity in patients with pulmonary disease or congestion.
[82Rb] images sometimes have excessive bowel radioactivity, which can make it difficult to interpret the inferior region. This excessive tracer accumulation is similarly observed using [201Tl] SPECT. This may be resolved by water intake. In clinical practice at
the authors’ facility, when a patient has strong bowel activity at rest imaging, the patient would have a cup of water and the resting study would be repeated.
the authors’ facility, when a patient has strong bowel activity at rest imaging, the patient would have a cup of water and the resting study would be repeated.
Assessment of Absolute Myocardial Blood Flow
Absolute measurement of physiological or biochemical function are obtained by tracer kinetics. A parametric physical model uses the time course of radioactivity of the arterial blood input function Ca(t), which usually can measure blood radio activity in the left ventricle (LV) cavity, and myocardial response function Cm(t) to estimate a quantitative rate constant (48,49). A simple net retention model can be used to calculate absolute MBF directly from short dynamic sequential images. The net retention equals the measured radio tracer concentration at time T divided by the integral of the blood input function curve to that time (50) or an earlier time point. The net retention reflects the effects of both MBF and cellular radiotracer extraction. This approach is mainly used for [13N]-tritium and [82Rb] blood flow quantification (22).
Compartment models have been developed for MBF quantification. The compartments represent tissue volumes that include physical factors (arterial blood and intracellular fluid) or biochemical factors (tracer compound and labeled metabolite). A mathematical model is constructed with parameters such as a flux of radioactivity between the compartments. The compartment model approach is mainly applied for [13N]-tritium and [15O]-water blood flow quantification (51,52).
Nitrogen-13-Ammonia Blood Flow Quantification
The most common approach assumes a three-compartment model with the compartments being the vascular, extravascular, and metabolic spaces (51). The first pass extraction is assumed to be 100%. K1 representing the transport of the tracer from the vascular space into the extravascular space is an estimate of MBF. These PET measurements have been well validated with microsphere measurements in experimental preparations confirming that regional MBF can be quantitatively measured over a wide blood flow range with [13N]-ammonia and this three compartmental approach (53,54,55). A number of simplified approaches have been used to quantify regional MBF with [13N]-ammonia. The simplest method is the microsphere model, which requires one static scan and arterial input function and correction for the net extraction fraction (50,56,57). However, the quantitative value is quite variable depending on the time of measurement after tracer administration. To minimize this effect, Patlak graphic analysis of the early uptake phase has recently been applied for the quantitative estimate of MBF (58,59).
Oxygen-15-Water Myocardial Blood Flow Quantification
Since water is freely diffusible in the myocardium without dependence on metabolism, the biological behavior of [15O]-labeled water can be modeled with a simple one-compartment model as originally described by Bergmann et al. (60) and Kety (61). Rapid sequential image acquisition is needed after tracer administration. Because of the short physical half-life, a large amount of activity is administrated that requires a high count rate and high sensitivity PET camera for quantification of radioactivity concentration after dead time correction.
Tomographic visualization of the [15O]-labeled water perfusion images requires correction of blood pool activity. A separate scan is acquired after inhalation of [15O]-carbon monoxide, which labels erythrocytes and delineates the vascular blood pool. High contrast myocardial perfusion images can be obtained after subtraction of the blood pool images from the [15O]-water images (Fig. 11.1.8) (60,62). Principal component analysis has been used to define the blood and myocardial signals, without the need for an additional blood pool scan. Patient motion between the two scans can introduce significant error into the subtraction images. The kinetic model used in the determination of MBF accounts for partial volume and blood-to-tissue spillover effects.
Iida et al. (52) proposed a mathematical model to correct the input function for the tissue-to-blood spillover (63) and partial volume effect (64). This model permits estimation of the perfusable
tissue fraction defined as the water perfusable tissue divided by total extravascular anatomical tissue. This parameter is independent of the size of the region of interest and may discriminate water perfusable viable tissue from nonperfusable infarcted tissue, assuming that irreversibly damaged tissue cannot exchange water rapidly (65,66).
tissue fraction defined as the water perfusable tissue divided by total extravascular anatomical tissue. This parameter is independent of the size of the region of interest and may discriminate water perfusable viable tissue from nonperfusable infarcted tissue, assuming that irreversibly damaged tissue cannot exchange water rapidly (65,66).
Manual positioning of regional myocardial regions of interest takes a long time and depends on the operator because [15O]-water uptake is not easily visualized as it is not retained in the myocardium. Katoh et al. (67) developed an automatic algorithm to calculate regional MBF using semiautomatic regions of interests setting algorithm and uniform input function. This approach could facilitate quantitative blood flow analysis using [15O]-labeled water PET in a clinical setting.
Hyperemic Myocardial Blood Flow and Coronary Flow Reserve
Hyperemic MBF represents microcirculatory vascular function, including both vascular smooth muscle and endothelial function. Vasodilatation is induced through both these mechanisms (68). Coronary flow reserve (CFR) is a ratio of near maximal MBF during pharmacologically induced hyperemia to MBF at rest. Thus, CFR represents coronary vascular function (5). The CFR measured by PET accurately reflects regional MBF as measured by intracoronary Doppler flow guide wire (69,70) and has good reproducibility using [13N]-ammonia or [15O]-water PET (71,72). Normal CFR measured using PET is 3.0 to 5.0 either by [13N]-ammonia, [15O]-water, or [82Rb] (Table 11.1.2). Dobutamine stress may have less increase in MBF: a 2.4-fold increase at peak dobutamine dose (40 μg/kg/min) (73). Adding atropine to the maximum dose of dobutamine can increase MBF comparable to dipyridamole stress (74).
Visual interpretation of relative tracer uptake both in PET and SPECT MPI is the standard approach for image interpretations. However, blood flow quantification seems to be more sensitive than the conventional approach (Fig. 11.1.9). Parkash et al. (20) evaluated the clinical importance of coronary flow reserve over conventional visual analysis in patients with three-vessel CAD using [82Rb] PET. The perfusion defect sizes were larger using quantification comparison with conventional relative uptake evaluation (69% ± 24% vs. 44% ± 18%; P = .008). Moreover, Yoshinaga et al. (75) compared the clinical value of MBF quantification and coronary flow reserve using [15O]-water PET with relative perfusion estimation using technetium-99m (99mTc) SPECT MPI. Segments with coronary stenosis without a perfusion abnormality observed by 99mTc SPECT MPI had reduced coronary flow reserve compared with remote segments (2.22 ± 0.87 vs. 2.92 ± 1.21; P <.02). These data indicate MBF quantification can add valuable information to conventional approaches. However, further investigations in a large study population are needed to fully understand the clinical utility and added value of this approach.
Endothelial Function
Coronary endothelial cells protect the coronary artery as mechanical barrier and through production of vasoactive factors, anticoagulant factors, and anti-inflammatory factors. Endothelial dysfunction is the earliest abnormality in the development of coronary atherosclerosis.
Coronary angiography or Doppler flow measurements during intracoronary administration of acetylcholine are the standard approaches for measurement of coronary endothelial function. Blood flow response is well correlated between intracoronary administration of acetylcholine and cold pressor test. The cold pressor test is also available for perfusion PET studies. It is now possible to define early stages of coronary atherosclerosis that
demonstrate endothelial dysfunction and vascular smooth muscle dysfunction measured as impaired coronary flow reserve or using cold pressor test or pharmacological vasodilator stress using adenosine or dipyridamole. MBF usually increases 30% to 60% of resting flow in normal volunteers (Table 11.1.3). PET MBF measurements during cold pressor test show high repeatability in both normal and smokers (r = 0.81; P <.0001) (76).
demonstrate endothelial dysfunction and vascular smooth muscle dysfunction measured as impaired coronary flow reserve or using cold pressor test or pharmacological vasodilator stress using adenosine or dipyridamole. MBF usually increases 30% to 60% of resting flow in normal volunteers (Table 11.1.3). PET MBF measurements during cold pressor test show high repeatability in both normal and smokers (r = 0.81; P <.0001) (76).
Table 11.1.2 Normal Values in Baseline Myocardial Blood Flow and Coronary Flow Reserve in Normal Subjects | |||||||||||||||||||||||||||||||||||||||||||||||||||||||||||||||||||||||||||||||||||||||||||||||||||||||||||||||||||||||||||||||||||||||||||||||||
---|---|---|---|---|---|---|---|---|---|---|---|---|---|---|---|---|---|---|---|---|---|---|---|---|---|---|---|---|---|---|---|---|---|---|---|---|---|---|---|---|---|---|---|---|---|---|---|---|---|---|---|---|---|---|---|---|---|---|---|---|---|---|---|---|---|---|---|---|---|---|---|---|---|---|---|---|---|---|---|---|---|---|---|---|---|---|---|---|---|---|---|---|---|---|---|---|---|---|---|---|---|---|---|---|---|---|---|---|---|---|---|---|---|---|---|---|---|---|---|---|---|---|---|---|---|---|---|---|---|---|---|---|---|---|---|---|---|---|---|---|---|---|---|---|---|
|
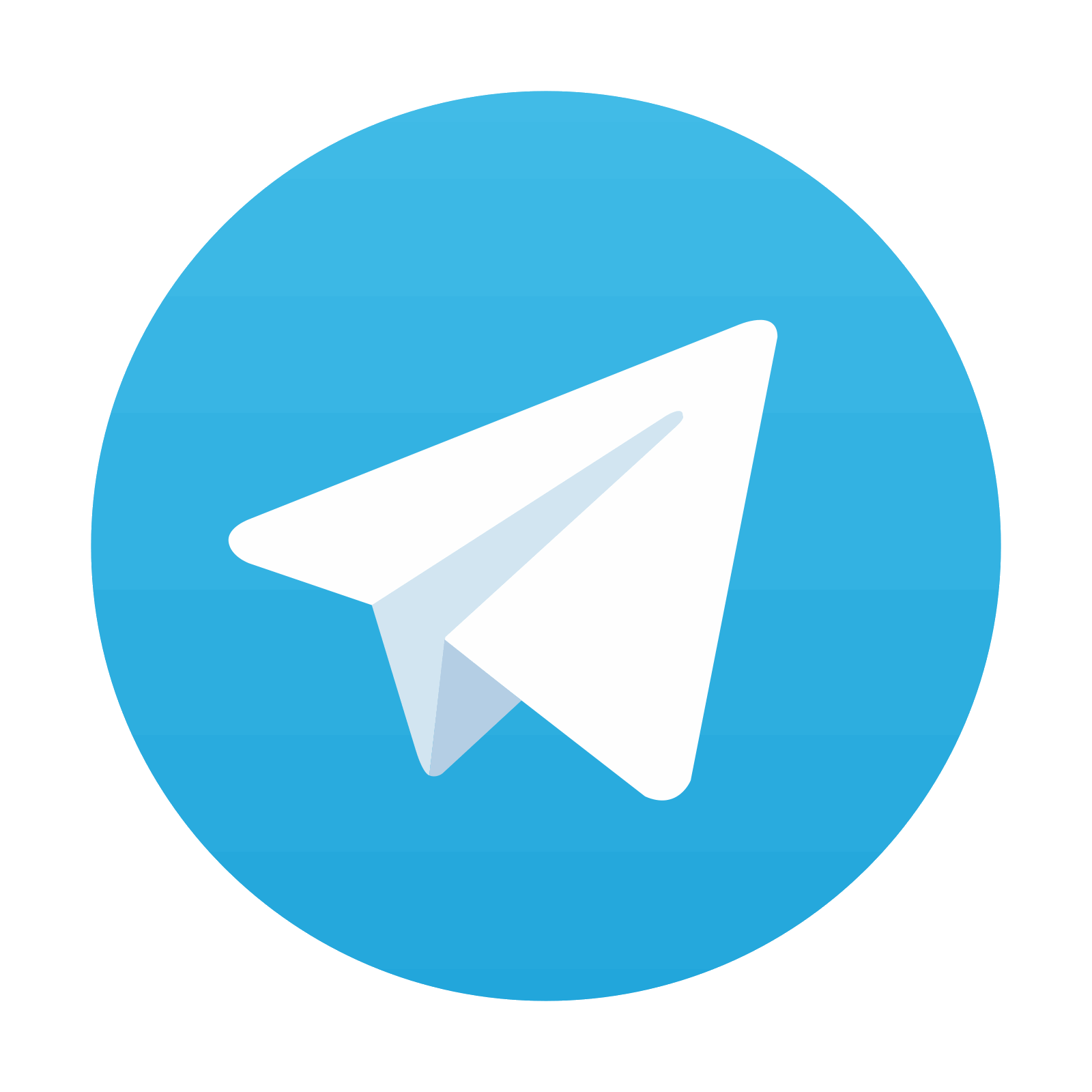
Stay updated, free articles. Join our Telegram channel
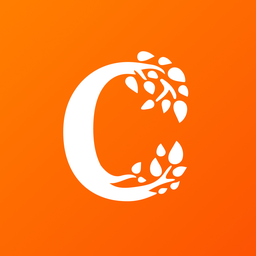
Full access? Get Clinical Tree
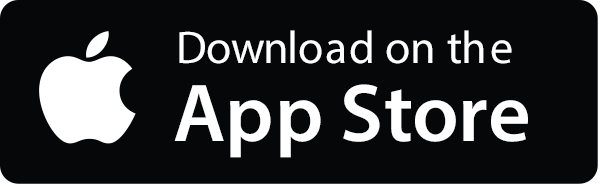
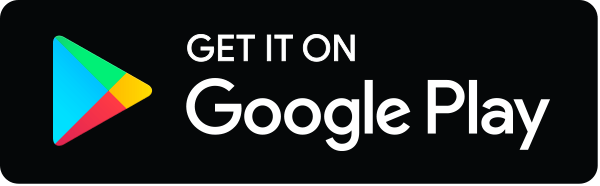