Functional Renal Anatomy, Renal Physiology, and Contrast Media
▪ FUNCTIONAL RENAL ANATOMY AND RENAL PHYSIOLOGY
The kidney maintains the homeostasis of body fluids through the excretion of metabolic end products and toxins, the regulation of body fluid volume and blood pressure, and the regulation of mineral and acid-base balance. Body fluids are divided into two discrete compartments. Approximately two-thirds of the fluid is located within cell membranes, which is termed as the “intracellular fluid.” The remaining one-third is contained in the extracellular compartment and called “extracellular fluid (ECF).” Approximately one-third of the volume of the ECF is contained within the vascular space and two-thirds in the interstitium. The regulatory function of the kidney directly affects the fluid that is located in the vascular compartment; however, because there is free movement of water between the intracellular and ECF compartments, the effect of this function is to regulate the composition of all body fluids.
Functional Renal Anatomy
The kidney consists of (1) an inner renal medulla and (2) an outer renal cortex (Fig. 4.1). The functional unit of the kidney is the nephron (Fig. 4.2). Each kidney contains approximately one million nephrons. Each nephron consists of a specialized capillary vascular network called the glomerulus, which is surrounded by Bowman capsule, a balloonlike structure into which the capillary tufts of the glomerulus protrude. Each glomerulus is connected to a series of specialized epithelial segments that collectively are known as the renal tubule.
The tubule, in turn, is divided into several segments. The first segment, the proximal tubule, is subdivided into convoluted and straight portions; the second segment, the loop of Henle, is subdivided into the thin descending, the thin ascending, and the thick ascending limbs; and the third segment, the distal tubule, is subdivided into the distal convoluted tubule and the cortical, medullary, and papillary collecting ducts. The cortex contains the glomeruli, the proximal tubule, the distal tubule, and the cortical collecting duct. The medulla is made up of the loops of Henle, the medullary and papillary collecting ducts, and renal pyramids, the apices
of which project into the minor calyces. The nephrons that are located close to the corticomedullary junction have larger glomeruli and their loops of Henle descend deeper into the renal papilla than those located more superficially in the renal cortex.
of which project into the minor calyces. The nephrons that are located close to the corticomedullary junction have larger glomeruli and their loops of Henle descend deeper into the renal papilla than those located more superficially in the renal cortex.
![]() FIGURE 4.1. Bisected section of the kidney showing the relationship of the cortex, the medulla, and the renal collecting system. Fat in the renal sinus surrounds the calyces and the renal pelvis. |
The main renal artery branches into interlobar arteries that divide into the arcuate arteries located at the corticomedullary junction. These, in turn, become the interlobular arteries that finally divide into the afferent arterioles, each of which leads to a glomerulus. The glomerulus is drained by an efferent arteriole that subdivides to form a peritubular capillary network known as the vasa recta. The vasa recta then anastomose to form venous channels. This unique arrangement in the kidney, in which the glomerulus is located between two resistive capillary networks as opposed to the arteriole-capillary-venule arrangement of the other tissues of the body, helps to maintain constant hydrostatic pressure at the level of the glomerulus despite changes in blood pressure (Fig. 4.3) and is the driving force for glomerular filtration.
The macula densa is a distinctive portion of the tubule between the ascending loop of Henle and the distal convoluted tubule that courses between the afferent and efferent arterioles. It represents the tubular component of a specialized area of the nephron called the juxtaglomerular apparatus. The juxtaglomerular apparatus is the site of renin synthesis and plays a major role in blood pressure regulation.
Basic Renal Physiology
The homeostatic functions of the kidney are achieved through two simultaneous processes: (1) glomerular filtration and (2) tubular resorption/secretion. Glomerular ultrafiltration occurs as a result of the net Starling forces across the glomerular capillary wall. The net filtration pressure (NFP) is equal to the sum of the glomerular hydrostatic pressure and the colloid osmotic pressure in Bowman capsule (which favors fluid movement from the capillary space into Bowman space) minus the sum of the mean glomerular capillary oncotic pressure and the hydrostatic pressure in Bowman space (the principal forces opposing ultrafiltration). Because protein is not filtered by the glomerulus, the fluid of Bowman space is protein free; thus, the colloid osmotic pressure in Bowman space is negligible. The glomerular filtration rate (GFR) is determined by the NFP and the surface area available for filtration, as well as by the permeability of the glomerular capillary bed. Thus, GFR can be expressed as
GFR 5 Kf × NFP,
where Kf is a constant expressing the product of the capillary permeability and the surface area of the capillary bed, and NFP is the net filtration pressure. The average GFR in a human is approximately 125 mL per minute, which is equal to 180 L per day or approximately 12 times the volume of the ECF.
Although 180 L of fluid is filtered in a day, only 1 to 2 L of urine is produced per day in which wastes and toxins may be concentrated 100- to 200-fold above their plasma concentration. This concentration of the final urine product is the result of tubular secretion and reabsorption. Under normal conditions, approximately two-thirds of the ultrafiltrate volume is reabsorbed by the proximal tubule by a process linked to the active secretion of hydrogen ions and the active reabsorption of sodium, glucose, amino acids, and other solutes. Isotonicity of the fluid in the proximal tubule with the plasma is maintained because the cells of the proximal tubule are freely permeable to water.
In the loop of Henle, which begins at the corticomedullary junction, differential absorption of sodium chloride occurs so that the fluid in the tubular lumen, initially isotonic with the interstitium, becomes progressively more concentrated in the descending limb, reaching its maximum concentration at the bend of the loop and then becomes progressively more hypotonic with respect to plasma as it reaches the thick ascending limb of the loop. This differential absorption of sodium chloride occurs because the cells in the descending limb have a high permeability for water, but a low permeability for salt; whereas, in the thick ascending limb, the cells are impermeable to water, but have a high permeability for salt, which is actively reabsorbed. This process, known as the
renal counter-current mechanism, results in progressive interstitial hypertonicity in the medulla and is required for final concentration of the urine by the distal tubules.
renal counter-current mechanism, results in progressive interstitial hypertonicity in the medulla and is required for final concentration of the urine by the distal tubules.
The distal tubule continues the water dilution of urine through the active transport of sodium and chloride coupled with relative water impermeability. The collecting ducts are the primary site of action of antidiuretic hormone (ADH). The final 15% of water absorption is achieved within the collecting ducts. The collecting ducts are virtually impermeable to water in the absence of ADH, but when ADH is present, water passes freely across the tubular wall, allowing the tubular fluid to achieve the same tonicity as the fluid in the surrounding interstitium. Thus, a hypertonic urine is produced without the active transport of water. The distal nephron also reabsorbs sodium and secretes hydrogen ions and potassium under the influence of aldosterone. Parathyroid hormone also acts on the distal tubule to conserve calcium.
Clearance
The rate at which a substance is removed from the plasma in a given period is termed as the clearance of that substance. For a substance that is freely filtered and not reabsorbed or secreted by the tubule, the rate of clearance is equal to the GFR. The rate of clearance is expressed as the urinary volume per unit time multiplied by the urine concentration of that substance divided by the plasma concentration of the substance. Thus
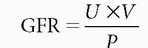
where U is the urine concentration of the substance, V is the urine volume produced per unit time, and P is the plasma concentration of the substance.
The polysaccharide, inulin, meets the criteria expressed above, and therefore, can be used to determine GFR. Creatinine, an endogenous product of muscle metabolism, is produced by the body in relatively constant amounts per day, is present in the plasma, and is excreted by glomerular filtration. Measurement of creatinine clearance is therefore convenient, although it is not as exact as the inulin clearance, because a small amount of creatinine is secreted by the tubules. Because creatinine clearance tends to underestimate renal dysfunction, particularly with mild impairment, the National Kidney Foundation has suggested that the estimated glomerular filtration rate (eGFR) may be a better model for predicting renal dysfunction than relying solely on serum creatinine determinations. This (eGFR) is generally calculated from the serum creatinine by either the Cockcroft-Gault formula or the modified diet in renal disease (MDRD) formula.
Renal Plasma Flow
If a substance was freely filtered and removed from the blood completely in one pass through the kidney, clearance of that substance should represent the renal plasma flow (RPF). Para-aminohippurate (PAH) is freely filtered and that portion that is not filtered is virtually completely secreted by the proximal tubule, so that at low plasma concentrations of PAH, all plasma supplying nephrons is completely cleared of the substance. The clearance of PAH, therefore, should represent the RPF; however, because 10% to 15% of renal blood flow supplies non-nephron-bearing portions of the kidney (i.e., the renal capsule and the peripelvic fat), the clearance of PAH is referred to as the effective renal plasma flow (ERPF). The normal value for ERPF is 650 mL per minute/1.73 m2 in males and 600 mL per minute/1.73 m2 in females. Once the ERPF has been calculated, the effective renal blood flow (ERBF) can be calculated by the formula:
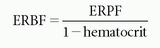
The filtration fraction is the ratio of the clearance of inulin to that of PAH or Cinulin/CPAH. In humans, this ratio is normally 0.2.
▪ CONTRAST MEDIA—HISTORICAL BACKGROUND
Although attempts at radiography of the urinary tract began shortly after Roentgen’s discovery in 1895, the first report of opacification of the urinary tract was in 1923, when researchers at the Mayo Clinic discovered opacification of the bladder in patients being treated with sodium iodide. It is remarkable that in the more than 80 years since this discovery, no element other than iodine has proven suitable for this purpose. Attempts at developing an injectable radio-opaque iodinated contrast medium were initially made in Germany under the direction of Moses Swick, an American urologist who was working in the laboratory of Professor Leopold Lichtwitz and focused on linking the iodine to the 5-carbon pyridine ring and subsequently to a 6-carbon benzene ring in an attempt to increase water solubility and decrease toxicity.
In 1955, Hoppe et al. at Sterling Winthrop Research Institute produced sodium diatrizoate (Fig. 4.4), a fully substituted derivative of 2,4,6-triiodobenzoic acid, which became the first modern ionic contrast medium. It was ionic in that the positively charged sodium (cation) dissociated from the negatively charged diatrizoate (anion) in solution. This product and its derivatives, including sodium/meglumine diatrizoate and meglumine iothalmate, became the standard urographic contrast media for the next 30 years.
Although these ionic contrast media were safer than the previously used materials, much of the remaining toxicity of the compounds was related to their high osmolality (>1,500 mOsm per kg of water or approximately five times greater than plasma). In 1968, Torsten Almen of Malmö, Sweden, a radiologist, theorized that the high osmolality of ionic contrast could be reduced by synthesizing a product that would be nondissociating. He suggested that the ionizing carboxyl group of conventional contrast be replaced with a nondissociating group such as an amide. This would theoretically decrease the osmolality by 50% by reducing the number of particles in solution without loss of iodine content. The first nonionic contrast medium, metrizamide, was never used in the United States as a urographic agent, because of its considerable cost and the necessity to package it as a freeze-dried lyophilized powder that needed to be reconstituted with water immediately before use.
Several years after the introduction of metrizamide, secondgeneration low-osmolality contrast media were introduced into clinical practice. These products developed along two general lines. In the first group, hydrophilic nonionizing radicals are introduced in positions one, three, and five of the benzene ring; whereas, positions two, four, and six remain the position of the iodine atoms. Because the number one position does not dissociate, the number of iodine atoms relative to the number of particles in solution is increased from 1.5 to 3 as compared with ionic contrast media. Compounds in this category include iohexol (Fig. 4.5; Omnipaque; GE Healthcare Inc., Princeton, NJ), iopamidol (Fig. 4.6; Isovue; Bracco Diagnostics Inc., Princeton, NJ), iopromide (Ultravist, Bayer Health Care, Wayne, NJ) and ioversol (Fig. 4.7; Optiray; Mallinckrodt Imaging, Hazelwood, MO). These products are generically known as nonionic monomers.
The second line of development of low osmolality contrast has been directed toward the linkage of two triiodinated benzene rings sharing one ionizing carboxyl group. These compounds have a ratio of six iodine atoms to two molecules in solution and also produce a ratio of 3. They are generally known as monoacidic dimers. The further development of this class of compounds involves the linkage of two nonionic monomeric compounds to form a nonionic monoacidic dimer, which has a ratio of six iodine atoms to one particle in solution, and therefore, represents ratio six compounds. This modification reduces the osmolality of the contrast medium close to that of plasma. Iodixanol (Fig. 4.8; Visipaque; GE Healthcare, Princeton, NJ), a ratio six dimer, is the first of the agents to be introduced into the United States.
▪ PHYSIOLOGY OF CONTRAST EXCRETION
Principles
The currently used triiodobenzoic acid derivative contrast media are excreted by glomerular filtration without significant tubular secretion. In 1967, Cattel et al. at St. Bartholomew’s Hospital in London demonstrated a direct relationship between the plasma concentration of contrast and the dose of contrast administered (Fig. 4.9). After intravenous injection of contrast medium, there is a rapid increase followed by a rapid decrease in plasma concentration of contrast. Although the majority (88%) of the rapid decline is due to equilibration of the contrast throughout the ECF, 12% of the decrease in contrast concentration due to excretion of the contrast by the kidneys represents the diagnostic opacification of the urinary tract during urography because it is during this period that glomerular filtration of the contrast medium is at its maximum (Fig. 4.10). It is therefore within the first few moments after injection that the nephrogram, representing contrast within the renal tubules, is at its peak intensity.
The degree of opacification of the urinary tract is not a function of the concentration of the contrast medium in the urine alone, but it is the total amount of contrast (urinary contrast concentration times the volume of urine produced) on which opacification depends. Thus, opacification depends on the total number of iodine atoms in the path of the X-ray beam rather than on their concentration in the urine. There is also a dose-related increase in the urine flow rate that occurs after contrast administration related to the fact that the contrast medium acts as an osmotic diuretic. However, the diuretic effect is greatly reduced with low osmolar and iso-osmolar contrast media.
As previously noted, the formula for glomerular filtration is

Opacification of the urinary tract can be described by rearranging the equation so that
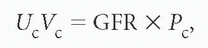
where Uc is the urinary contrast concentration, Vc the volume of urine produced, Pc the plasma concentration of contrast, and GFR the patient’s glomerular filtration rate. The product, UcVc,
therefore, represents the total amount of contrast medium in the urine The intensity of the nephrogram is directly related to the amount and concentration of contrast given and the speed with which it is administered. Although the rate of excretion of contrast medium is greatest in the first 10 minutes after injection, it falls logarithmically thereafter. With increasing time, the contrast that had equilibrated with the ECF returns to the vascular space and is excreted. Approximately 24 hours is required to excrete 100% of the administered dose (Fig. 4.11).
therefore, represents the total amount of contrast medium in the urine The intensity of the nephrogram is directly related to the amount and concentration of contrast given and the speed with which it is administered. Although the rate of excretion of contrast medium is greatest in the first 10 minutes after injection, it falls logarithmically thereafter. With increasing time, the contrast that had equilibrated with the ECF returns to the vascular space and is excreted. Approximately 24 hours is required to excrete 100% of the administered dose (Fig. 4.11).
The sequential change in the computed tomography (CT) attenuation value for the renal cortex that occurs after bolus administration of contrast medium intravenously directly correlates with the amount of iodine administered. Indeed, a plot of CT number against time (Fig. 4.12) produces a curve similar in appearance to the plot of plasma contrast concentration against time. Therefore, the change in CT number in the renal cortex following contrast administration accurately reflects the physiology of contrast excretion.
Physiologic Considerations
In the past, overnight fluid restriction was often recommended to improve the diagnostic quality of excretory urography. The rationale of this approach was based on the physiologic principle that in a dehydrated state, ADH production is stimulated and results in more concentrated urine. However, a lower urine volume is produced. As overnight fluid restriction is ineffective in producing a
statistically significant change in preinjection urinary osmolality and attempted dehydration may pose an increased risk to the patient, particularly, the so-called high-risk debilitated patient or the patient with multiple myeloma, fluid restriction before contrast administration is not recommended.
statistically significant change in preinjection urinary osmolality and attempted dehydration may pose an increased risk to the patient, particularly, the so-called high-risk debilitated patient or the patient with multiple myeloma, fluid restriction before contrast administration is not recommended.
Overhydration may have an adverse effect on pyelographic density in patients being hydrated with intravenous fluids. A “washout” of the pyelogram can occur, but the quality of the nephrogram is usually unaffected. The ideal patient for urography, therefore, is one in a state of euhydration.
Phases of Contrast Enhancement
The greatest single factor affecting the quality of the urogram is the dose of contrast administered. On CT, three distinct phases of contrast enhancement can be identified: (1) a bolus phase (an attenuation difference of 30 Hounsfield units [HU] or greater between the aorta and the inferior vena cava); (2) a nonequilibrium phase (aortocaval difference of between 10 and 30 HU); and (3) an equilibrium phase (aortocaval difference of <10 HU). Studies have suggested that enhancement and thus tissue differentiation are greatest in all three phases for body CT when the contrast medium is administered as a bolus rather than by infusion technique. Three distinct phases of contrast enhancement of the kidneys can be seen after a bolus of contrast medium has been administered by a mechanical injector: these are termed as (1) the corticomedullary phase (15 to 60 seconds after injection), (2) the nephrographic phase (80 to 120 seconds), and (3) the excretory phase (>120 seconds).
Extrarenal (Vicarious) Excretion
In patients with normal renal function, less than 1% of a dose of injected contrast medium is excreted via a nonrenal route. The primary routes of nonrenal excretion are through the biliary tract and the small bowel. In normal circumstances, this excretion is not detectable on plain radiographs, even when a high dose of contrast medium is employed. Contrast may be visible normally in the gallbladder on CT scans obtained 15 to 48 hours after a large dose of contrast medium is administered. This visibility is presumed to be related to the high contrast sensitivity of CT and is not a manifestation of renal or hepatic disease.
In patients with depressed renal function, however, excretion of the contrast via the biliary and small bowel routes is
markedly increased and may be visible on plain films. Such excretion has been termed vicarious excretion. The exact mechanism for this phenomenon is not certain, but it is speculated that in such patients protein binding of the contrast medium occurs and results in increased hepatic excretion. As a corollary, contrast administration is believed to be contraindicated in patients with hepatic and renal disease, because both the hepatic and the renal routes for excretion may be impaired. In addition to biliary excretion, there is evidence that there is direct transmural excretion of contrast through the small bowel. Such excretion is usually not visible until the contrast reaches the colon, where water absorption increases the concentration of the contrast medium. Direct colon excretion of the contrast medium is not thought to occur. There has been a concern that the administration of contrast media to patients with little or no renal function might have adverse hemodynamic effects, and therefore, immediate hemodialysis after contrast administration should be instituted. However, it has been shown that dialysis is not necessary and that even functionally anephric patients can be given nonionic contrast media without discernible effects. The contrast so administered will be excreted through biliary and small bowel routes.
markedly increased and may be visible on plain films. Such excretion has been termed vicarious excretion. The exact mechanism for this phenomenon is not certain, but it is speculated that in such patients protein binding of the contrast medium occurs and results in increased hepatic excretion. As a corollary, contrast administration is believed to be contraindicated in patients with hepatic and renal disease, because both the hepatic and the renal routes for excretion may be impaired. In addition to biliary excretion, there is evidence that there is direct transmural excretion of contrast through the small bowel. Such excretion is usually not visible until the contrast reaches the colon, where water absorption increases the concentration of the contrast medium. Direct colon excretion of the contrast medium is not thought to occur. There has been a concern that the administration of contrast media to patients with little or no renal function might have adverse hemodynamic effects, and therefore, immediate hemodialysis after contrast administration should be instituted. However, it has been shown that dialysis is not necessary and that even functionally anephric patients can be given nonionic contrast media without discernible effects. The contrast so administered will be excreted through biliary and small bowel routes.
▪ CONTRAST MEDIA—PHYSICAL PROPERTIES
A variety of physical and chemical properties may be used to describe the contrast media in general use in the United States. With the older, ionic contrast media, the strength of the contrast is frequently expressed in terms of percent concentration. This number represents the number of grams of contrast per 100 mL of water. For example, Hypaque 50 (GE Healthcare, Princeton, NJ) is 50 g of sodium diatrizoate in 100 mL of water. This designation does not, per se, describe the amount of iodine in solution; but in general, the higher the percent concentration, the more radiopaque the contrast medium. Some contrast media use the numerical designation to denote the amount of iodine in milligrams per milliliter of the contrast medium. Thus, Conray 325 (Mallinckrodt Imaging, Hazelwood, MO) contains 325 mg of iodine per mL of solution.
The “osmolality” of a contrast solution is equal to the number of particles in the solution per kilogram of water. It is determined by the concentration of contrast medium and the molecular size of the compound. Thus

where N is equal to the number of iodine atoms per molecule, 127 is the molecular weight of iodine; K is the number of particles in solution, and G is the osmotic coefficient of the specific media that varies with concentration and molecular size. Because K is equal to 2 for ionic contrast media and equal to 1 for nonionic contrast media, one can readily determine that the nonionic agents have approximately one-half the osmolality of ionic agents at equal iodine concentrations. The osmolality of differing contrast media must be compared with one another at equal iodine concentrations, because the lower the iodine concentration, the lower the osmolality. In general, the larger the molecular weight of the agent, the lower the osmolality.
Another physical property of contrast media is viscosity. This property describes the relative adhesiveness of the molecules of the contrast medium for one another and is important because the viscosity of the contrast determines how rapidly the contrast may be injected. The viscosity of contrast media decreases with increasing temperature. The unit of viscosity is the poise; the commonly used unit is the centipoise, which is equal to 0.01 poise.
▪ ADVERSE REACTIONS TO CONTRAST MEDIA
Reactions to contrast media, although uncommon, continue to constitute a significant hazard to patients despite considerable research into their nature, incidence, and mechanisms. Minor side effects including flushing, a metallic taste in the mouth, and tachycardia are common; they usually resolve within a few minutes and should not be considered reactions in the usual sense.
Adverse effects of contrast media can be divided into two groups: (1) idiosyncratic or anaphylactoid reactions, those which mimic an allergic response to contrast media, and (2) chemotoxic effects, those thought to be secondary to a direct toxic effect of the contrast media. Because many of the reactions to contrast media mimic those produced by known allergens (i.e., urticaria or bronchospasm) but are not mediated by immunoglobulins, these reactions are sometimes referred to as “anaphylactoid.”
▪ IDIOSYNCRATIC REACTIONS
Idiosyncratic reactions are generally classified as (1) mild, (2) moderate, and (3) severe. Mild reactions are defined as those having only minor effects requiring no therapy. Moderate reactions are those that are transient and not life-threatening, but usually require therapy. Severe reactions are defined as those that are life-threatening and that require intensive therapy. The symptoms of these reactions are listed in Table 4.1.
TABLE 4.1 Symptoms of Contrast Material Reactions | ||||||||||||||||||||||||||||||||||||||||||||||||
---|---|---|---|---|---|---|---|---|---|---|---|---|---|---|---|---|---|---|---|---|---|---|---|---|---|---|---|---|---|---|---|---|---|---|---|---|---|---|---|---|---|---|---|---|---|---|---|---|
|
Large clinical trials have demonstrated significantly fewer adverse reactions to the intravenous administration of nonionic contrast media than conventional ionic contrast media. Katayama evaluated more than 300,000 patients who received intravenous contrast media for various examinations in Japan and estimated that nonionic media are safer by a factor of 6 compared with conventional contrast. In another study, Palmer found that adverse reactions occurred five times more commonly in low-risk patients who received ionic media compared with those who received nonionic contrast. An overall adverse reaction rate of 0.6% to nonionic contrast has been reported from the University of Michigan in a series encompassing more than 80,000 contrast injections for CT over a 6-year period.
Risk Factors
Factors that predispose a patient to developing an idiosyncratic reaction to contrast medium have been extensively studied (Table 4.2). In general, patients at greatest risk are in the older age groups and have significant preexisting disease.
Patients with a history of allergy to food, drugs, and ragweed (i.e., hayfever) or asthma have an increased chance of having a contrast reaction, but the magnitude of this increased risk varies somewhat in the reported series.
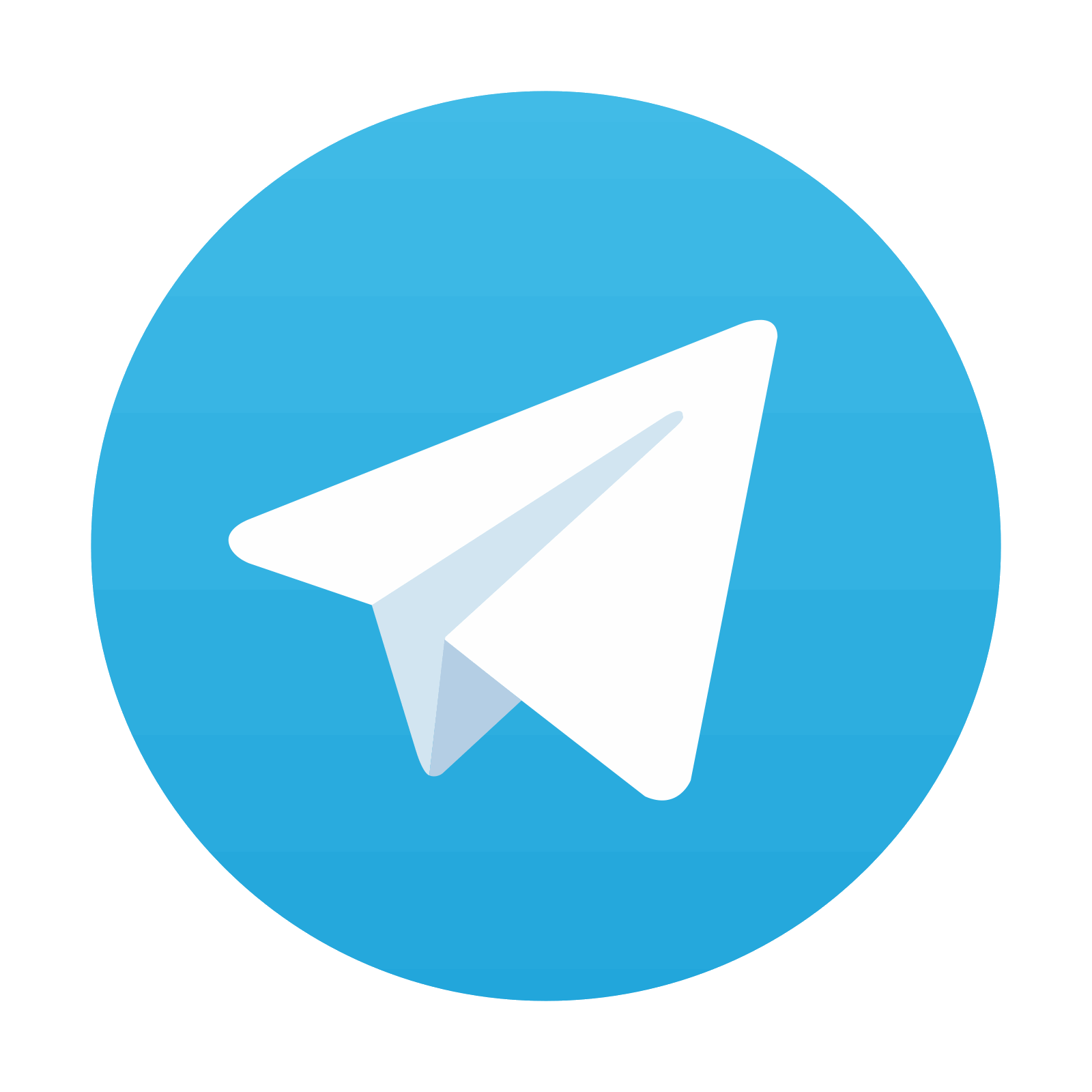
Stay updated, free articles. Join our Telegram channel
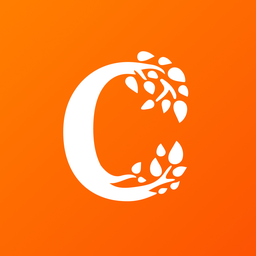
Full access? Get Clinical Tree
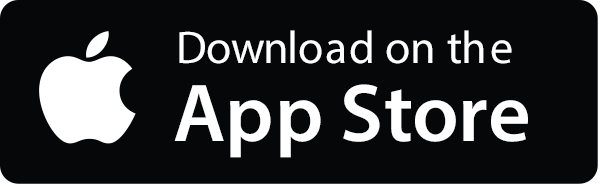
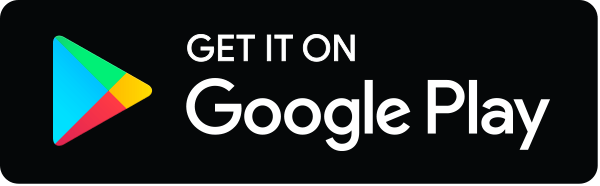