Fig. 33.1
Neurobiopsy from rear of scanner. (a) Neurosurgeon easily reaches into short-bore MR scanner to align trajectory guide for MR-guided neurobiopsy. (b) Oblique sagittal turboFLAIR MR image shows needle tip within suspected thalamic glioma. Note artifact identifying needle tip. (c) Oblique coronal turboFLAIR MR image confirms needle is indeed within, not overlying, tumor (Courtesy C. Truwit, MD Minneapolis, MN)
At the Brigham, neurosurgeons could perform a craniotomy while literally standing inside the MR scanner. In Minnesota, two surgical theaters were available: at the rear of the scanner inside the 5 Gauss (5G) line and at the front end, well outside the 5G line. The Minnesota investigators’ notion was that the patient could be advanced out the back end of the scanner just enough such that the patient’s head would be accessible to the surgeon. Initially, the architecture allowed for 40 cm of extension beyond the bore of the scanner [12]. In this way, both minimally invasive procedures and open craniotomy would proceed in much the normal fashion, constrained only by limitations related to the table and the need for MR-compatible surgical cutlery. The patient’s surgical field (i.e., the head) could be placed at isocenter to obtain pre-procedure, intradissection, and post-procedure images. In this manner, an approach quite similar to that of the Brigham and Women’s hospital would be possible, albeit at high-field strength. This concept would reveal itself to be ideal for minimally invasive procedures such as neurobiopsy was and feasible for open craniotomy.
The Minnesota suite also included the ability to operate just beyond the 5G line, in which case patients would be placed on a pseudo-surgical table (modified angiography table) that could be rotated in such a manner that all draping, instrumentation, and the microscope could be safely deployed outside the 5G line and, when appropriate, removed such that the patient could be pivoted back into alignment with the MR scanner couch. With appropriate surgical draping, the patient could be shuttled into the scanner for imaging (Fig. 33.2). In addition, a modest degree of Trendelenburg positioning could be achieved which was not possible inside the bore, even if the patient were extended 40 cm beyond the bore opening.
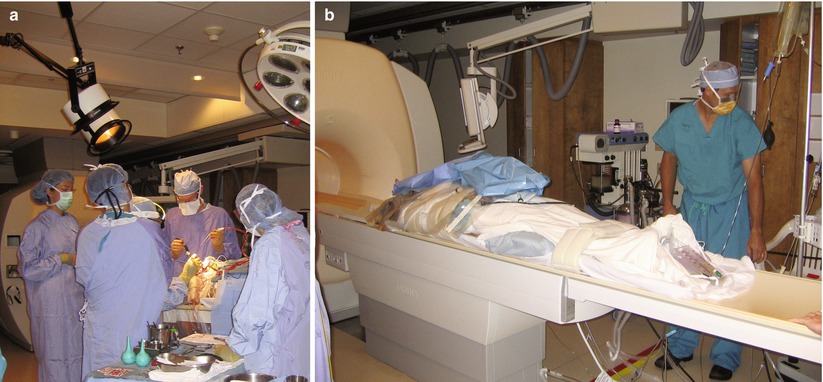
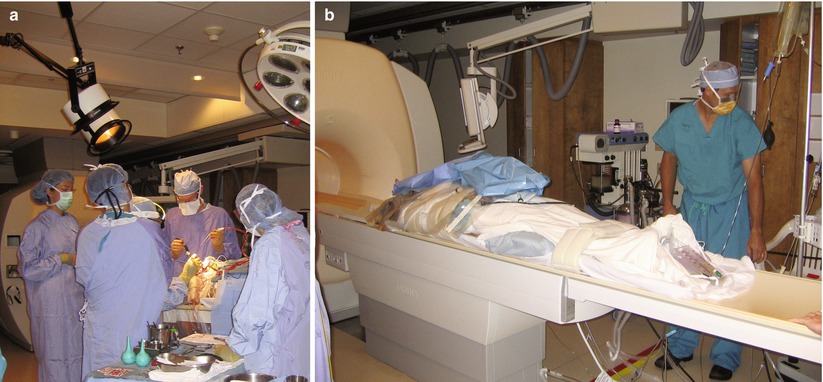
Fig. 33.2
Shuttle patient from outside 5G. (a) Neurosurgery performed outside the 5G line. (b) Surgical draping adjusted to allow for maintenance of sterile field. Tabletop (with patient) is being shuttled into scanner for intradissection MR imaging (Courtesy C. Truwit, MD Minneapolis, MN).
Though the “rear” approach had its many advantages, ultimately the “inside the fringe field” approach practiced in Minnesota would prove to be too far removed from current conventional neurosurgical practice for many surgeons to embrace. Instead, they would opt for Minnesota’s other surgical approach (outside the 5G line) or, alternatively, for the Calgary approach (move the magnet), or a dual room approach with a MR-operating room (OR) trolley to shuttle patients from the OR to the MR. While more cumbersome than at the rear of the scanner, these other approaches proved to be less disruptive to – and therefore, more acceptable to – neurosurgeons wanting to undertake iMR-guided neurosurgery.
Thus, for many tumor resections, surgeons preferred having access to the scanner while operating outside the 5G line. This approach was rapidly adopted by other centers. The group at Case Western Reserve University (Cleveland, OH) worked with Siemens Medical Systems (now Siemens Healthcare Solutions, Erlangen, Germany) to develop a pivoting, tilting, and canting MR surgical table [13]. This elegant solution was implemented at Case as well as at the University of California Los Angeles and the University of Erlangen, where neurosurgeons successfully operated immediately outside the 5G line.
While investigators in Minnesota and at other sites were comfortable with the concept of shuttling their patients into and out of the scanner during surgery, investigators in Calgary approached this issue in a different manner. The Calgary group felt strongly that moving the patient was not optimal and proposed to move the scanner. Working with image-guided magnetic resonance imaging systems (IMRIS, Winnipeg, CA), the Calgary team found a solution to moving the magnet (Fig. 33.3). Suspended from two rails, the magnet was moved easily from a parked position into the suite, literally enveloping the patient and surgical table. Initially, this transport proved to be costly in time, but eventually, the system proved both versatile and appealing to neurosurgeons who envisioned the MR scanner as a step up from intraoperative US and wanted to be able to update their frameless neuronavigation data sets.
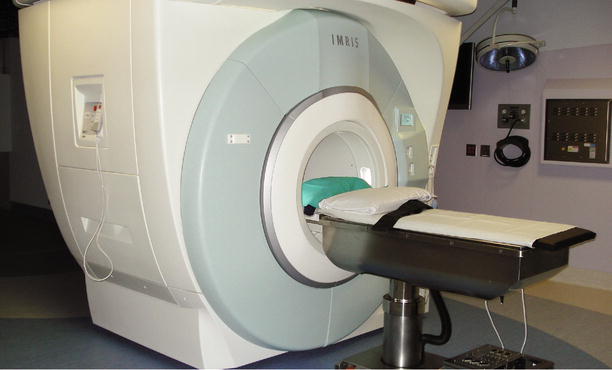
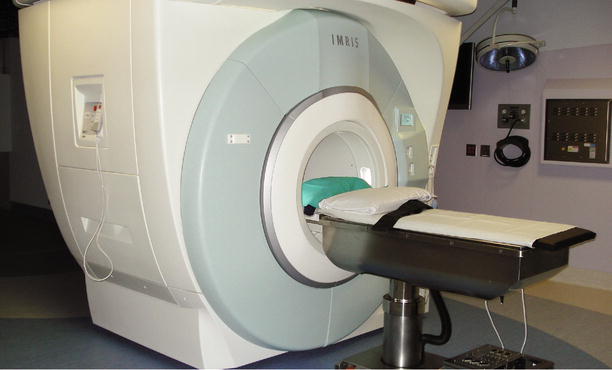
Fig. 33.3
IMRIS system with mobile MR scanner and fixed surgical table (Courtesy M. Nagib, MD Minneapolis, MN)
As has been described extensively in the literature, investigators were initially focused on the issues of brain shift and the completeness of resection. At the Brigham, surgeons had clearly demonstrated the value of intradissection imaging to identify residual tumor after the neurosurgeon had determined the resection to be complete, by his or her visual inspection of the tumor bed [2]. In fact, the pioneering work at the Brigham revealed to the neurosurgery community at-large that what exceptionally competent neurosurgeons were used to declaring total resection was, in fact, incorrect, with residual tumor revealed by intradissection imaging in the majority of cases. Additionally, what would be confirmed quite rapidly at the Brigham and all other sites was that brain shift was not only quite real and rate-limiting in many cases of tumor resection, but that it could be addressed easily by the addition of intradissection MR imaging. Surgeons could “update” their frameless stereotaxy data sets in order to be able to continue to use the frameless systems safely throughout the surgical procedures.
These stunning revelations spurred great interest in iMR-guided neurosurgery as a useful adjunct to optimize conventional tumor resection. Indeed, if these were to be the only gains, many surgeons would argue that this was value enough to advance their field, akin to the introduction of the neurosurgical microscope [14].
Move the Patient, Park the Scanner
The Minnesota suite was first opened in late 1996 and included several unique features. The room was longer than the typical MR suite, housing not only the MR scanner but a surgical zone outside the 5G line; a second surgical pit behind the scanner, well within the 5G line; and a C-arm fluoroscopy unit which could be used at the conventional end of the room for angiography and/or fluoroscopy. As noted above, the Minnesota team envisioned performing several types of procedures in its suite, including conventional neurosurgery for tumor resection, minimally invasive neurosurgery from neurobiopsy to placement of neurostimulators for Parkinson’s disease, and stroke therapy including intra-arterial thrombolysis under both catheter and MR guidance. Other, non-neurosurgical procedures were also envisioned and performed but are beyond the scope of this chapter.
Initially, the University of Minnesota suite was utilized for a series of carotid artery balloon test occlusion procedures, principally as a dry run for potential iMR-guided intra-arterial thrombolysis of patients suffering from acute stroke [15]. For various reasons, the concept of endovascular stroke therapy under MR guidance has been touted by many, yet successfully performed by few. Whether the potential benefits of monitoring intra-arterial thrombolysis with advanced MR diffusion/perfusion imaging are real or perceived, the difficulties of such work in an emergent situation have to date proved more problematic than anticipated. Thus, while a dozen endovascular balloon test occlusion cases were successfully performed under MR guidance in Minnesota (Fig. 33.4), the cerebrovascular goals of iMR-guided therapy have to date remained elusive.
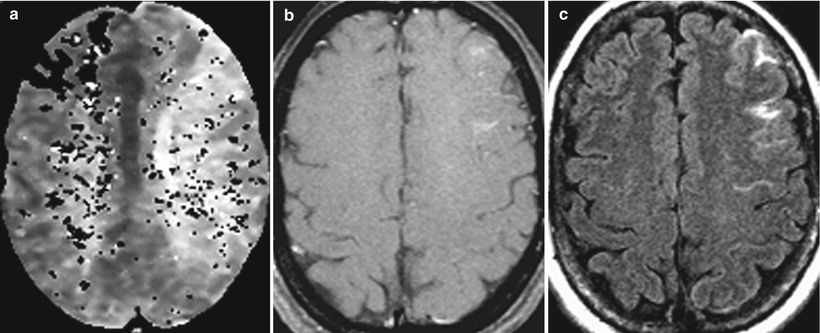
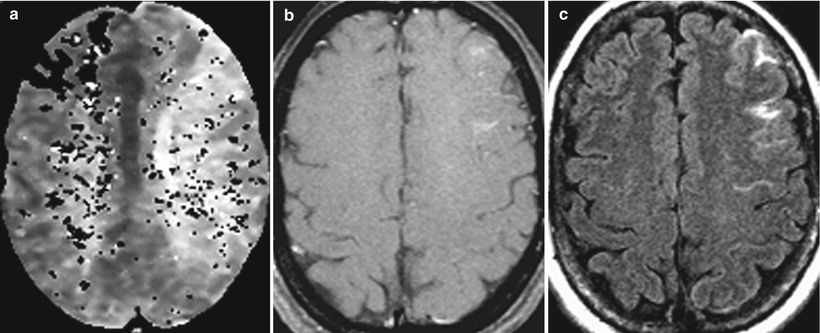
Fig. 33.4
Internal carotid artery balloon test occlusion. Initially, patient appeared to tolerate the test occlusion but developed symptoms at 27 min. (a) Axial susceptibility-weighted perfusion MR shows delayed perfusion to left middle cerebral artery territory. (b) Axial post-contrast T1-weighted image shows mild leptomeningeal enhancement. (c) Axial FLAIR post-contrast image shows more prominent leptomeningeal enhancement. This was the first study to show the value of contrast-enhanced FLAIR imaging, in this case for the detection of cerebrovascular ischemia (Courtesy C. Truwit, MD Minneapolis, MN)
The Minnesota group undertook its first neurosurgical procedure in February 1997, successfully performing a freehand brain biopsy without complication. Certainly, there can be debate about the merits of freehand neurobiopsy versus stereotactically guided neurobiopsy. Nevertheless, the early cases in Minnesota confirmed the benefit of intratumoral needle placement under direct MR-visualization and the intraoperative imaging demonstration of the absence of complications.
These early cases confirmed the feasibility of this type of work at higher-field strength. Whereas doubts had been raised about the potential of iMR at 1.5T simply due to the anticipated problems with imaging artifacts due to magnetic susceptibility, the Minnesota work showed convincingly that such artifact could be contained. Moreover, work by the Minnesota group and others revealed that manipulating the gradient read and phase directions in conjunction with alignment of the needle parallel to the main magnetic field (B0) could optimize the needle shaft and needle tip artifact such that the needle could be easily visualized and its tip position verified (Fig. 33.5) [16, 17].
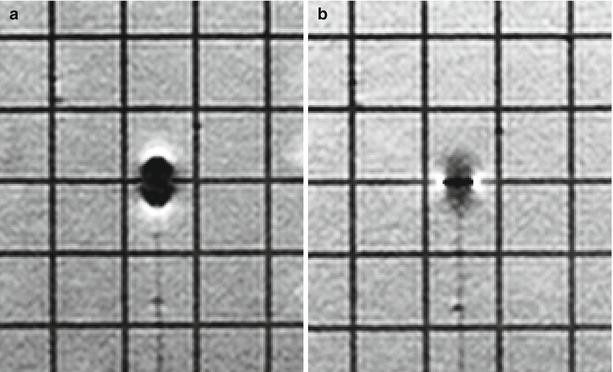
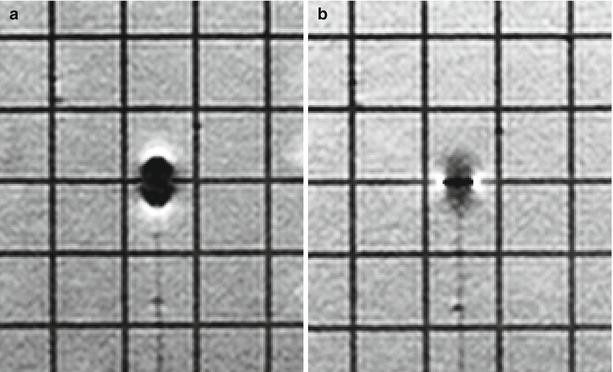
Fig. 33.5
Needle artifact. Note differences in degree and type of artifact. In both images, the needle is aligned along B0. In (a) the read gradient is aligned parallel to the needle shaft. Blooming of the needle tip makes it difficult to precisely identify its location. Needle shaft is barely visible. In (b) the read gradient is still aligned parallel to the needle shaft, although polarity of read gradient has been reversed. Needle tip is well visualized and can be precisely determined (between white artifacts). See Fig. 33.1 (b, c): read gradient is aligned largely along the plane of the needle, polarity reversed (Courtesy C. Truwit, MD Minneapolis, MN)
Freehand biopsy was not a forward-looking concept, and to appropriately optimize the procedure, a new approach to guidance would be necessary. Traditionally, such guidance took the form of framed stereotaxy and, subsequently, frameless stereotaxy. Various implementations of both approaches had been developed and were well accepted by neurosurgeons. In general, the state of the art in the late 1990s was such that framed stereotaxy was used by functional neurosurgeons and neurooncologists for neurobiopsy. Frameless stereotaxy, on the other hand, was used for craniotomy guidance, neurobiopsy, and to some degree “picket-fencing” around tumors prior to, and in an attempt to address, intraprocedural brain shift.
Both framed and frameless approaches were based on prior imaging, and although many investigators had envisioned using iMR imaging to update the data sets that form the imaging basis behind these approaches, as of the 1990s, the concept of using direct image guidance was still unclear. To address this, two principal approaches emerged: the first was the SLICER developed at MIT in conjunction with investigators at the Brigham and Women’s Hospital and is described in Chap. 22 [18]. The SLICER was a brilliant concept of rapid updating a prior data set by means of a series of orthogonal localizing scans. Such “refresh” localizers could be performed repeatedly in very short order and would both keep the validity of the original 3-dimensional (D) data set and with mathematical modeling would allow for data set morphing to accommodate 3-D brain shift.
The second approach, prospective stereotaxy, was developed in Minnesota [19]. Prospective stereotaxy entailed the collinear alignment of three points to secure a surgical trajectory. Drawing a line from the intended target outward through a pivot point at the skull opening and into the space beyond, surgeons could perform alignment by plotting against a plane perpendicular to the intended path of navigation. Invented with prospective stereotaxy was an MR-compatible ball-and-socket device (Navigus, Medtronic-IGN, Melbourne, FL) with an MR-visible stylus. Once aligned, the ball would be locked, the stylus removed, and a needle or electrode introduced under direct visualization of orthogonal imaging planes along the needle. In this manner, an MR-compatible needle could be seen by its “negative” as it traversed through the brain and entered a brain tumor for purposes of neurobiopsy [12] or accessing a craniopharyngioma cyst (Fig. 33.6) [20]. Further developments included MR spectroscopically guided and remote-controlled brain biopsy [21, 22] and radiofrequency ablation of the cingulate gyri and placement of depth electrodes (Truwit CL, unpublished data).
The Navigus was one of three devices invented to solve trajectory guidance through a burr hole. Another, the Snapper-Stereo-Guide (MagneticVision, Zurich, Switzerland) used frameless stereotaxy for guidance and was used largely at GE Signa SP sites [23]. The third such device, the NeuroGate (Daum, Schwerin, Germany), was made of titanium and was used primarily at lower-field strengths due to its metal artifact [24]. Like the Snapper-Stereo-Guide, the Navigus was made of MR-compatible medical grade plastic.
In San Francisco, investigators built on the work in Minnesota [25]. Again, burr holes were placed outside the 5G line; the patient was passed through the scanner where he or she was redraped before reintroduction into the scanner. In Minnesota, a stereotactic head frame was used. In San Francisco, the NextGen Navigus was secured to the burr hole. Typically, the patient would be centered with a z-direction offset to allow for the Navigus stem to be included within the field of view. Using prospective stereotaxy, the NextGen Navigus stylus was aligned with the imaging-derived subthalamic nucleus targets. With the system locked and the trajectories confirmed by post-alignment orthogonal scans, the neurostimulators were introduced (Fig. 33.7). Post-placement images confirmed appropriate positioning of the leads. This work has been advanced elsewhere confirming that DBS placement can be performed successfully under iMR guidance and without microelectrode recording [26, 27].
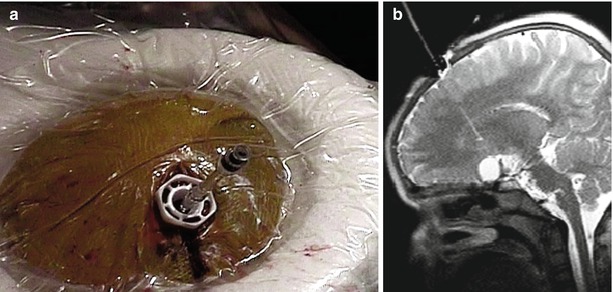
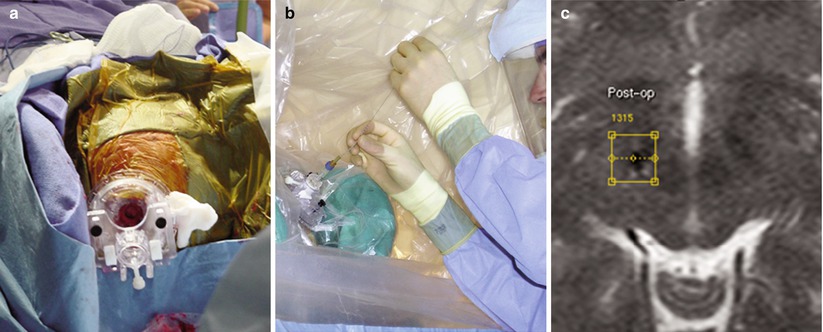
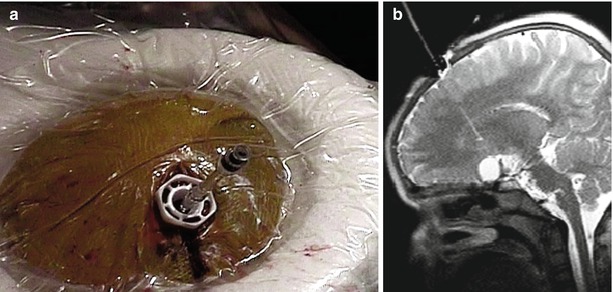
Fig. 33.6
Navigus stylus. (a) Navigus with saline-filled stylus is secured into burr hole within center of sterile-prepped radiofrequency coil. (b) Sagittal T2-weighted image shows craniopharyngioma cyst, linear signal within brain from prior stereotactic-frame based attempt to reach cyst, and alignment of Navigus stylus in preparation for puncture of cyst to deliver therapeutic dose of radioactive P-32 (Courtesy C. Truwit, MD, Minneapolis, MN)
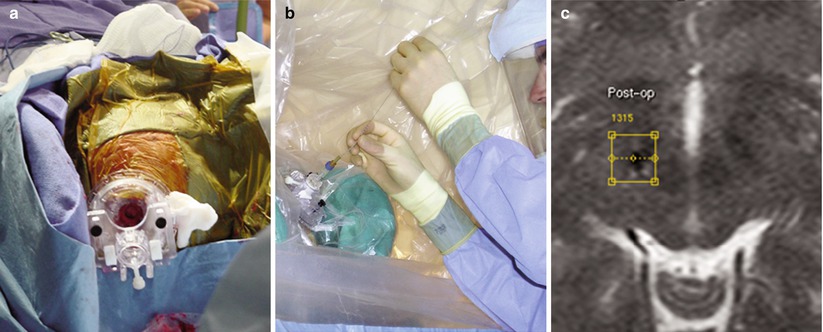
Fig. 33.7
NexGen trajectory guide (Medtronic-IGN, Fridley, MN). (a) Skull-mounted trajectory guide. (b) Neurosurgeon leans into draped end of MR scanner to introduce neurostimulator. (c) Post-placement T2-weighted image confirms susceptibility artifact from lead and overlay of pre-placement target (yellow diamond within yellow square) (Courtesy Alastair Martin, PhD, San Francisco, CA)
More recently, MR Interventions (formerly Surgivision, Irvine, CA) has introduced the ClearPoint system (Fig. 33.8). While similar to the NextGen Navigus, the system has a few differences and offers a more holistic approach, including not only the skull-mounted navigation device and guidance software, but also a dedicated multichannel radiofrequency head coil. The guidance software is a variant of traditional frameless stereotaxy systems. Early indications are that its guidance accuracy approaches that of prospective stereotaxy [28].
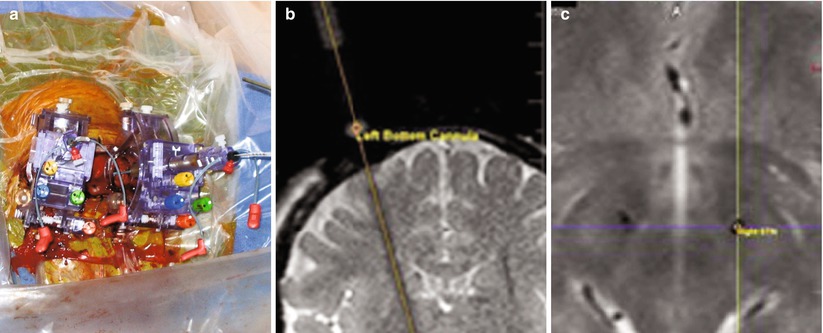
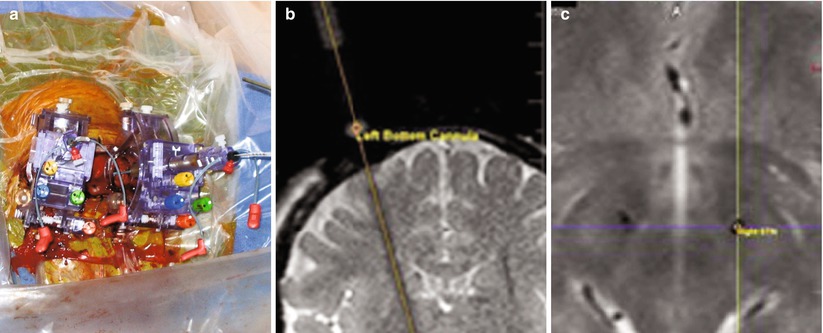
Fig. 33.8
Clearpoint surgical trajectory guidance (MRI Interventions, Irvine, CA). (a) Skull-mounted trajectory guide. (b) MR-visible stylus is aligned along intended trajectory on oblique coronal T2. Oblique sagittal image (not shown) confirmed trajectory alignment. (c) Post-implantation axial T2-weighted image confirms proper placement of neurostimulators within subthalamic nuclei (Courtesy A. Martin, PhD, San Francisco, CA)
While neurobiopsy and minimally invasive functional neurosurgical procedures could thus be optimized using iMR-guided neurosurgery, investigators of high-field iMR initially sought to address the question of optimizing tumor resections. As noted above, the early work at these sites focused on the questions of identifying the presence of residual tumor and compensating for brain shift. Additionally, it would become routine to perform scans dedicated to searching for intraoperative hemorrhage with gradient echo scans and stroke with diffusion-weighted images. Rapidly, the imaging was augmented with MR venography to delineate surface vasculature prior to craniotomy, with preoperative functional MR imaging to define eloquent cortices, with MR spectroscopy to better characterize the metabolic signatures of lesions prior to surgery, and, most importantly, with diffusion tensor imaging to provide white matter fibertracking. Initially, the fMRI and DTI were obtained preoperatively, either in the iMR-guided surgery suite just prior to anesthesia or potentially days earlier.
Thanks initially to the work of Nimsky et al. in Erlangen, and others elsewhere, both fMRI and DTI subsequent to the induction of anesthesia and during the acquisition of not only preoperative [29], but intradissection images [30] were able to be obtained (Fig. 33.9). This capability would again offer a quantum leap for neurosurgeons. Rapidly, electrical cortical mapping on awake patients in the MR-OR would, when combined with fMRI and DTI, offer the potential to undertake resection of tumors situated in close proximity to the motor strip [31–33]. Advancing the earlier work of Bernstein in Toronto [6], Nimsky in Erlangen [30], and others [30–32], Sawaya’s group at the MD Anderson Cancer Center in Houston, TX, included the switch from bipolar to monopolar activation to undertake resections of tumors as close a 5 mm from the motor strip with limited, if any, morbidity [34].