

even 230 to 270 mL for the intracranial spaces (18). This is an important concept because it implies that, just by pushing the brain to fill the pericerebral spaces, the ventricular volume may increase from 25 to 150 mL and more without changing the brain volume (a mathematical simulation of this process yields similar results) (19). The CSF is composed of water (99%), ions (Na+, K+, Ca++, Mg++, Cl−, HCO3−), proteins, amino acids, creatinine, urea, lactate, phosphate, and glucose (20,21); protein concentration is higher in newborns (especially those born preterm) than in older children, while glucose concentration is slightly lower (22). CSF serves as a transport vehicle for movement of various neurotrophic substances, signaling molecules, neurotransmitters, and other metabolites, to and from the brain. The concentration of these substances in the CSF is “analyzed” throughout life by the circumventricular organs, portions of the ventricular walls that are devoid of CSF-brain and blood-brain barriers and, thereby, allow the hypothalamus to react in response to changing CSF composition (23,24).
does not contain arachnoid septations (27). The dorsal attachment of the tela choroidea to the dura forms a transverse membrane that separates the cisterna magna from the retrovermian cistern (28). Conventionally, the dorsal aperture is called the foramen of Magendie and the openings of the lateral recesses into the cerebellopontine angles, the foramina of Luschka. The choroid plexus is attached transversely between the lateral recesses, along the inferior medullary velum: it is actually located in the cisterna magna as much as in the fourth ventricle. The caudal apex of the fourth ventricle is continuous with the (usually virtual) central canal of the caudal medulla and spinal cord and is covered by the obex; this is where the area postrema (most caudal circumventricular organs) lies.
might play a role in the expansion of the cerebral ventricles by maintaining an optimal hydrostatic pressure (43). This could also result from their arterial pulsatile force (see below). Although histologically similar, the choroid plexuses present different and specific molecular secretion patterns (“secretome”) in the lateral, as compared with the fourth, ventricles that are consistent with correspondingly specific forebrain and hindbrain receptors (21).
or produced by the epithelium; this “secretome” is both regionalized (different in the forebrain and the hindbrain, adapted to specific receptors in both locations) and evolutive according to the stage of brain development (21).
of CSF or venous drainage […] are blocked, the intraventricular pulse is increased correspondingly and this entire force must be absorbed by the brain” (6). This dual dampening process constitutes the compliance of the system. The loss of elasticity results in less attenuation of the force of the pressure wave, so that this force is fully exerted against the brain tissue. The dampening effect is also lost if the venous pressure is increased; again, the force of the pressure wave is exerted against the parenchyma. In both instances, a progressive loss of brain tissue may ensue. Modern studies have shown that the pulsatile force originates not from the plexuses only, but from the whole intracranial vascular system (pericerebral, intracerebral, and choroid) (8,12,69,93,94). They have emphasized its importance in the CSF hydrodynamics (7,9,11,93,95,96,97,98,99,100,101), and MR imaging has become a major investigation tool in this regard (10,93,94,99,101). Most of the force of the systolic inflow is buffered by displacing blood along the vascular system (94%); capillary and venous resistance therefore are potentially major factors of hydrocephalus (Table 8-1). Significantly smaller oscillatory volumes of CSF are displaced by the residual vascular expansion out of the ventricles and out of the cranium toward the more elastic spinal dural sac; in normal conditions, they have been evaluated at 1.7 mL/min at the aqueduct (with peak velocities of 10 cm/s, however) (10,11,102), 14.5 mL/min at the incisura, and 39 mL/min in the upper cervical canal (10) (Fig. 8-2). These movements produce the flow void artifacts that are easily detected on T2SE WI (103). In comparison, the amount of fluid displaced through the absorption sites is negligible: 0.35 mL/min, which is a hundred times less than the CSF volume displaced at C2-C3 (Table 8-1; Fig. 8-2). The prominent flow voids in the partially enclosed prepontine, suprasellar, and sylvian cisterns (104,105) also likely reflect local turbulences, probably due to basilar and carotid/sylvian arterial pulsations. The lack of an aqueductal flow void in normal neonates (personal data) can be explained by the elastic sutures/fontanels allowing the pulsating force to be fully centrifugal.
Table 8-1 Relationships of Blood and CSF Flows | ||||||||||||||||||||||||||||||||
---|---|---|---|---|---|---|---|---|---|---|---|---|---|---|---|---|---|---|---|---|---|---|---|---|---|---|---|---|---|---|---|---|
|
and in the closed cranium. The stability of a mass on the ground does not rule out the force of gravity.

consistent clinical finding is an excessive rate of head growth. An enlarging head circumference in a child is not, by itself, of concern (as it actually allows the preservation of brain volume), but an excessive rate of head growth (as compared with normal standards) demonstrated by serial head circumference measurements should raise the clinical suspicion of a developing hydrocephalus. In infants and young children, macrocrania is nearly always found at presentation. The forehead is disproportionately large (frontal bossing), the skull is thin, the sutures may be separated, the anterior fontanelle may be tense, and the scalp veins often are dilated. Ocular disturbances are frequent and include paralysis of upward gaze (“setting sun” appearance), abducens nerve paresis, nystagmus, ptosis, and diminished pupillary light response. Spasticity of the lower extremities is common, resulting from disproportionate stretching and distortion of the corticospinal axons that arise from the medial parts (leg area) of the motor cortex, which are more directly exposed to the compression and stretching by the dilated lateral ventricle than the more lateral corticospinal and corticobulbar axons that supply the upper extremities and the face. Although occasional cases of arrested hydrocephalus have been documented, a slow, progressive deterioration is more usual. An acute decompensation also may occur, with rapidly increasing ICP (Table 8-2). Factors that relate to the prognosis of hydrocephalus are the age of the patient at the onset of hydrocephalus and the duration of the disease (younger age and longer duration imply worse prognoses), in addition to the underlying cause. The development of a high ICP is also associated with poor prognosis.
Table 8-2 Clinical Classification of Obstructive Hydrocephalus | |||||||||||||||||||||||||||||||||||
---|---|---|---|---|---|---|---|---|---|---|---|---|---|---|---|---|---|---|---|---|---|---|---|---|---|---|---|---|---|---|---|---|---|---|---|
|
Three-dimensional, high-resolution (millimetric) T1 imaging with reformatting in orthogonal (or any appropriate) plane illustrates the location and severity of ventriculomegaly in addition to the general brain morphology.
Coronal T2-FSE demonstrates the rounding of the lateral ventricles, especially the temporal horns with the medial compression of the hippocampus, as well as stretching of the septum pellucidum (which is sometimes torn, especially in congenital hydrocephalus) and, posteriorly, of the hippocampal commissure (which often is displaced to run vertically instead of the usual horizontal orientation). The evaluation of ventricles (size and shape) for comparative follow-up studies should be made in this (coronal) plane by
measuring the longest transverse diameter of the atrium of the largest lateral ventricle. We prefer this measurement to the Evans’s index (generally used in adult hydrocephalus) because the ventricular dilatation in pediatric hydrocephalus is typically more prominent in the posterior part of the hemispheres due to the vulnerability of the white matter during the brain development (118,119) and, possibly, because the thin parietal bones allow greater ventricular expansion.
Sagittal thin T2-FSE images best demonstrate the gross morphology of the midline structures and the major ventricular and cisternal CSF flow voids. The corpus callosum and septum pellucidum are stretched, and the fornix is displaced inferiorly and separated from the splenium by the distorted (verticalized) hippocampal commissure. The anterior third ventricle demonstrates rostral bulging of the lamina terminalis, along with widening of the supraoptic and infundibular recesses, and flattening or inferior convexity of the normally superiorly convex tuber cinereum. The suprapineal recess may be prominent also, but the extent varies with individual anatomy. The aqueduct is well demonstrated; its appearance varies with the location and severity of the obstruction: sometimes normal-looking, sometimes compressed, sometimes occluded with or without proximal widening, or (often) dilated. The aqueductal CSF flow void must be evaluated on this sagittal T2-FSE sequence, as it becomes more prominent when either the velocity or the amplitude is increased (103,120). Two factors may, singly or in association, increase the amplitude of the pulsatile movements of CSF in the aqueduct: one is the effacement of the pericerebral space, which redirects the arterial pulsations medially; the other is the decrease of compliance that results in an increased P2 peak of the pulse wave, as discussed previously. In contrast, a flow void is rarely seen in normal young infants with a large elastic pulsatile fontanelle and a small spine because pulsations are directed centrifugally (from the center of the brain to the periphery). Finally, sagittal T2-FSE images beautifully show the fourth ventricle, the cisterna magna, and the posterior fossa cisterns along with prominent, basilar artery-related signal voids in the prepontine cistern. Obviously, this sequence may also show a midline mass in the suprasellar, velum interpositum, quadrigeminal, fourth ventricular, or retrocerebellar regions.
High-definition, thin (submillimetric) sagittal steady-state T2 imaging (FIESTA/CISS) images are extremely important in the assessment of hydrocephalus, providing clear images of thin membranes in the ventricles, aqueduct, or cisterns; it is especially important for presurgical planning of a third ventriculostomy in order to determine the patency of the interpeduncular cistern. Postventriculostomy, together with sagittal T2-FSE, CISS demonstrates both the anatomic (patent ventriculostomy) and the functional (transventriculostomy flow void) results of the procedure. It may also reveal cisternal obstructing membranes that were not apparent before the ventricles were decompressed.
Axial FLAIR sequences show the axial ventricular morphology and the parenchyma. In hydrocephalic patients with a high ICP, the ependymal indentation of the stretched subependymal veins and bright signal in the periventricular and deep white matter suggest subependymal venous compression and lack of ISF absorption in the territory of the deep medullary tributaries. In contrast, periventricular bright FLAIR signal in chronic hydrocephalus suggests defective myelination, secondary to the particular vulnerability of the oligodendroglial precursors (118). The two should not be confused.
Although it is generally considered that T2-FSE sequences demonstrate the aqueductal flow void as well as phase-contrast CSF-flow imaging, several dedicated sequences have been promoted to enhance the demonstration of the CSF flow: steady-state free precession (121); spatial modulation of magnetization (SPAMM) (122); reversed fast imaging with steady-state precession (PSIF) (123); 3D sampling perfection with application-optimized contrast (3D-SPACE) (124). The most recent, a time-spatial labeling inversion pulse (Time-SLIP) technique uses spin labeling of the water molecules to show CSF oscillation (125), but their rapid diffusion outside the area of interest may somewhat blur the picture. Dynamic cardiac-gated cine phase-contrast imaging is another way of demonstrating the flow; it is very popular as it allows one to quantify the CSF motion in groups of subjects or patients (10,126). Special techniques that take advantage of the relative phase angle changes of moving spins can be used to quantify the flow of CSF through the foramina of Monro, aqueduct, foramen of Magendie, or brainstem cisterns (Fig. 8-5) (10) and have been proposed for the functional assessments of the shunts. CSF motion, however, is so dependent upon so many different factors that the reliability of the measurements in individual patients is uncertain.
Other techniques. Diffusion tensor imaging (DTI) analyzes the effects of microstructural changes in the parenchyma upon water motion (mean diffusivity [MD], fractional anisotropy [FA]); it may demonstrate a periventricular stretching of the white matter tracts (127). 1H MR spectroscopy can be used to identify changes in the energy metabolism (128). In arterial spin labeling (ASL) perfusion imaging, the blood-water itself is labeled by the MR sequence and serves as an intrinsic contrast medium that is used to investigate the blood flow noninvasively (89,129). Pseudocontinuous ASL (pCASL) is currently considered to be the most accurate ASL technique in children but, in newborns and young infants, the very short distance between the site of labeling in the neck and the site of imaging in the brain, in addition to the rapid heart rate of very young babies, makes its utility rather variable. Positive contrast cisternography or ventriculography have been suggested and are considered safe (130) but have failed to gain real acceptance as the trend in brain imaging is toward minimal invasiveness; its additional value is questionable given the versatility of MR. MR venography (MRV) may demonstrate an acquired or developmental venous compromise, primary or secondary to the hydrocephalus. Other sequences that may assist in the etiologic assessment are susceptibility imaging (blood residues), diffusion imaging (abscess, epidermoid cyst), or postcontrast T1 (leptomeningeal enhancement).
“Fast” MR scanning is a technique that uses single-shot, ultrafast imaging sequences. Just like a “quick” CT, it alleviates the need for sedation/anesthesia and is often used in our practices instead of CT because of mounting concerns regarding the use of ionizing radiation in the follow-up of hydrocephalus in children (see Chapter 1) (131). These heavily weighted T2 sequences are adequate for assessing the ventricular/cisternal size and morphology and to show the position of the shunt, but they have poor sensitivity and specificity for parenchymal changes (Fig. 8-6).
much longer than in the mature brain, which implies that, in TSE/FSE sequences, both T1-weighted and T2-weighted the TR should be much longer; for T2 images, the TE should be lengthened as well. FLAIR sequences are essentially noncontributory in the setting of hydrocephalus. The immature calvarium is quite expandable due to the fontanelles and thin bones; with the small size of the spinal canal relative to the skull, the CSF displacement by the arterial expansion at systole is directed peripherally (pulsations of the fontanelle) rather than caudally, so that there is no CSF movement and, consequently, no flow void within the aqueduct of a newborn infant (Fig. 8-7). Due to the incomplete development of the subcortical white matter, the field of the deep medullary tributaries of the subependymal veins appears more extensive across the thickness of the cerebral mantle relative to the territory of the subcortical veins, compared with adults; therefore, periventricular interstitial edema appears more extensive than in a mature brain, and there is greater diffusivity of the interstitial water. This periventricular edema may be more difficult to recognize against the background of high parenchymal water content on T1WI and T2WI images. Also, fibrotic changes in the ventricular walls allow better appreciation of a periventricular rim of low signal contrasting with the bright signal of both the CSF and the white matter on T2WI. Finally, because of the characteristics of hydrocephalus in infants, the use of CISS/FIESTA to demonstrate arachnoid septations, or susceptibility-weighted sequences to demonstrate blood residue is particularly useful in the assessment of the hydrocephalic brain in this age group.
coil is placed in such a way that the fetal head is in the center of the field explored by the coil. Each sequence serves as the localizing scout for the next one. Slices should be 2 to 3 mm thick and cover the whole fetal brain in the three orthogonal (sagittal, coronal, and axial) planes.
Mass effects responsible for the hydrocephalus are easily recognized, and contrast enhancement helps in the characterization of the pathology. However, the diagnostic yield and the versatility of CT are much less that those of MR, and CT can expose children to significant doses of ionizing radiation (see Chapter 1). Therefore, in our practices, initial evaluation of the brain is based on MR, as it is fast, is accurate, gives off no ionizing radiation, and is equally (or more) sensitive to small narrowings in the CSF pathways. If CT is to be used for follow-up, the implementation of a low-dose technique actually limits evaluation mostly to assessment of ventricular size (Chapter 1).
![]() Figure 8-7 Midline sagittal T2-FSE in a neonate. Because of the large, open fontanelles, the parenchymal systolic CSF pulsations are directed outward; as a result, no flow void is seen within the aqueduct (white arrow, compare with Fig. 8-4B). |
![]() Figure 8-8 3D-CT imaging. Nice demonstration of the ventricular and cisternal anatomy in the axial (A), sagittal (B), and coronal (C) planes. Although not as sensitive and versatile as MR, modern CT is a fast and efficient diagnostic tool, and it is commonly used for screening or follow-up. Its main disadvantage is the radiation dose delivered, especially concerning in children. In most instances, a very low-dose CT (see Chapter 1) is used to check ventricular size. |
sonography may allow assessment of ICP noninvasively. Taylor and Madsen (137) studied the hemodynamic response to fontanelle compression in infants with ventriculomegaly, and used Doppler sonography to determine resistive indices in the anterior and middle cerebral arteries in premature neonates who had suffered intracranial hemorrhage. Baseline resistive indices without fontanelle compression did not correlate with ICP. However, a significant correlation was found between the changes in resistive index during fontanelle compression in children with elevated ICP, with the maximum change in resistive index significantly higher in infants who subsequently required shunting than in infants who did not (p = 0.001) (137). Thus, the need for shunt placement may be determined by assessment of resistive indices with and without fontanelle compression.
Table 8-3 Imaging Characteristics of Hydrocephalus (Given in Order of Utility) | ||
---|---|---|
|
or convex downward. This may compromise the hypothalamic nuclei and fascicles in the infundibulum and diminish the flow within the hypothalamic-pituitary portal venous system along the stalk, resulting in hypothalamic-pituitary dysfunction. When the aqueduct or the lumen or the outlets of the fourth ventricle are occluded, the tuber cinereum is characteristically hugely dilated and bulges into the interpeduncular cistern (Figs. 8-9A and B and 8-13A) sometimes wrapping the head of the basilar artery. The pulsating dilated anterior third ventricle may be so huge as to erode the dorsum sellae (Figs. 8-9A and 8-13A). When hydrocephalus is severe and chronic, the floor of the third ventricle may even rupture spontaneously, creating an internal drainage pathway that allows CSF to escape from the ventricular system into the subarachnoid space (143). The disproportionate enlargement of the recesses of the third ventricle results from the relatively small resistance to expansion provided by the thin hypothalamus and the cisterns that surround the walls of the recesses. In contrast, the thalami, which form the walls of the posterior third ventricle, provide a great deal more resistance to expansion. It is important to note that even with a significant ventricular enlargement the lateral walls of the third ventricle (i.e., the thalami) remain parallel to each other, but the massa intermedia becomes elongated and thinner (Fig. 8-9E).
On axial images, the dilated anterior recesses of the third ventricle are best detected by noting that the third ventricle is larger at the level of the optic chiasm (Fig. 8-13B) than at the level of the thalami: a rounded, circular posterior third ventricular lumen would indicate the presence of a cyst, usually a suprasellar cyst with superior expansion (Fig. 8-14). The normal suprapineal recess may be small or prominent depending on the individual anatomy; a dilated suprapineal recess of the third ventricle may sometimes expand into the posterior incisural space, displacing the pineal gland inferiorly and, occasionally, elevating the vein of Galen. Further posterior enlargement of the pineal recess may compress the tectum from the posterior direction, resulting in thinning of the tectum and narrowing of the aqueduct (144). As a rule, the anterior (supraoptic and infundibular) recesses seem to enlarge earlier and to a greater degree than the posterior (suprapineal) recess. Caudally, the dilatation may involve the proximal aqueduct with consequent shortening of the tectum in the rostral-caudal direction (144) (Fig. 8-13A). The short, thick tectum should not be mistaken for a neoplasm; isointensity with normal brain tissue on T2/FLAIR images and the absence of contrast enhancement exclude a tumor.
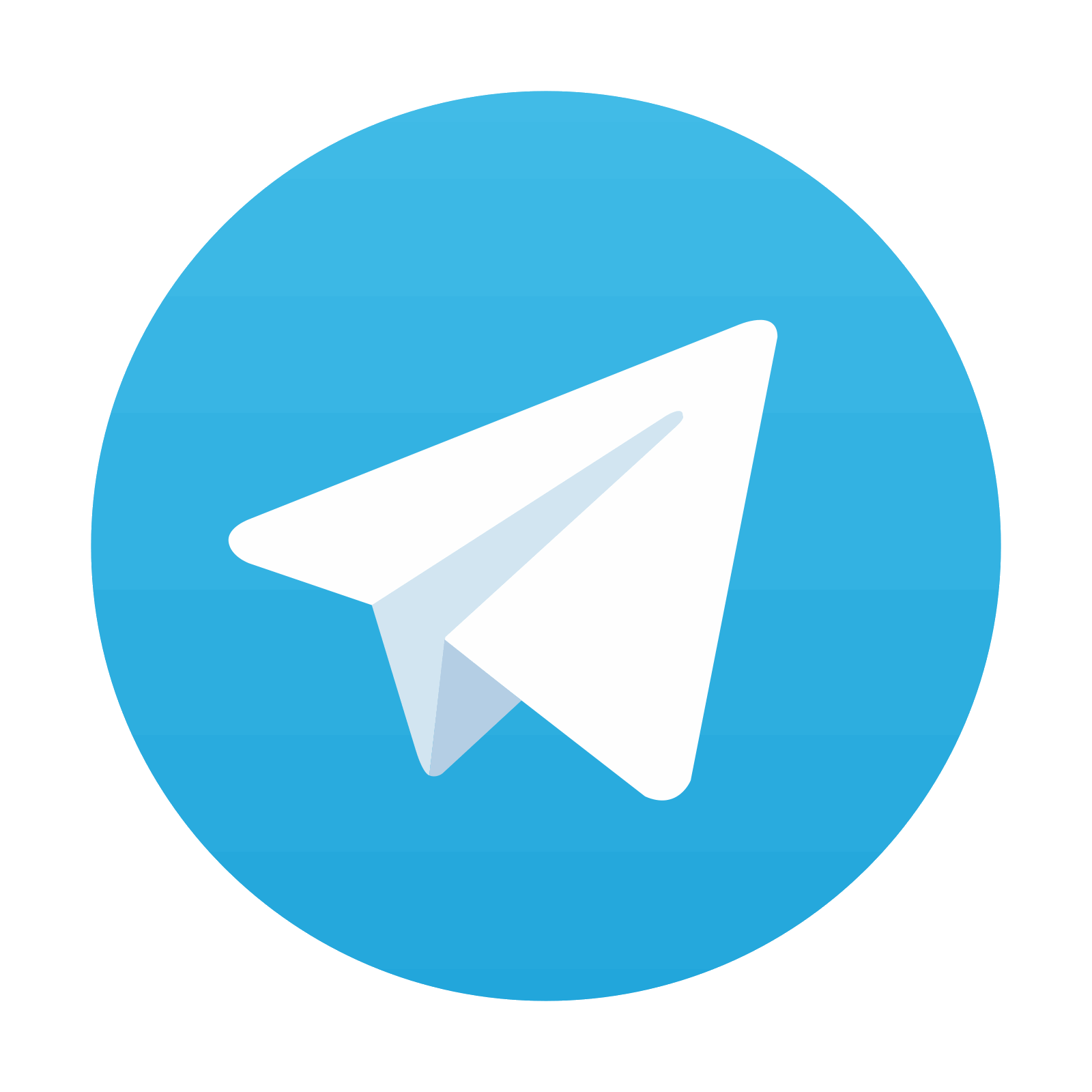
Stay updated, free articles. Join our Telegram channel
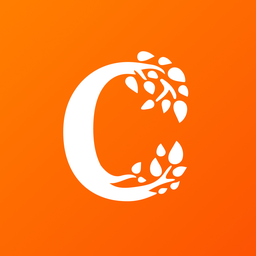
Full access? Get Clinical Tree
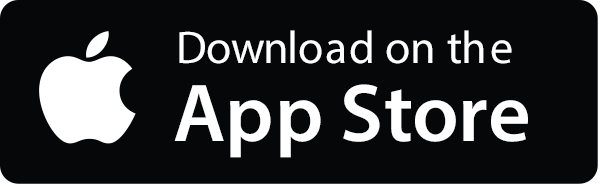
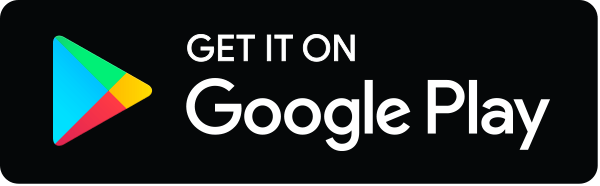
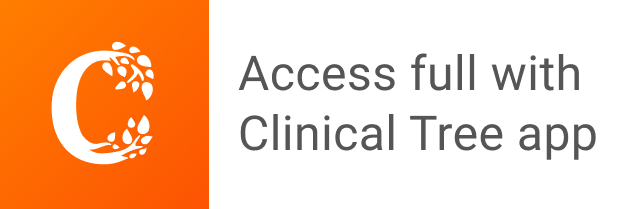