Hypoxia Imaging
Morand Piert
Definition of Hypoxia
A continued source of molecular oxygen is essential for cellular respiration and energy supply. Hypoxia is defined as a metabolic state in which the concentration of oxygen is below physiological levels (normoxia) but above the complete lack of oxygen (anoxia). Even under physiologic conditions, tissue oxygenation levels may vary considerably between different tissues and may display a marked heterogeneity even in tissues that are considered well perfused (1). Hypoxia should be differentiated from ischemia, the latter describing a lack of blood flow to a particular organ or tissue. Although ischemia can be caused by prolonged severe tissue hypoxia and by anoxia, these terms are clearly not interchangeable.
Cellular Regulation of Oxygen Homeostasis
The transcription factor hypoxia-inducible factor 1 (HIF-1) is a key regulator of hypoxia-induced gene expressions. Since the discovery of the HIF-1 system by Semenza and Wang (2), HIF-1 has been of particular interest for cancer biologists. HIF-1 is a heterodimeric protein that is composed of two polypeptides, the HIF-1α and HIF-1β subunits (3,4). With the completion of the human genome project, two additional HIF α members, the closely related HIF-2α (5,6) and more distantly related HIF-3α (7), were identified, although their function is less well defined.
The phosphatidylinositol 3-kinase (PI3K) and ERK mitogen-activated protein kinase (MAPK) pathways regulate the HIF-1α protein synthesis (8). HIF-1 activity is regulated at the posttranscriptional level by protein degradation of HIF α subunits (9). Under normoxic cellular conditions, HIF-1α is targeted by the oxygen-dependent prolyl hydroxylase to undergo ubiquitylation by E3 ubiquitin-protein ligases. These ligases contain the von Hippel-Lindau (VHL) protein.
Ubiquitylated HIF-1α is rapidly degraded by the proteasome (8). By contrast, under hypoxic cellular conditions, the prolyl hydroxylation is inactive and the HIF α members are not complexed with VHL and remain available in the cell. As a result, HIF-1β binds to the HIF-1α subunit, leading to the transcriptional activation of multiple genes responsible for cellular proliferation and cell survival, apoptosis, oxygen and nutrient delivery (angiogenesis), and anaerobic energy metabolism (glucose metabolism), all of which are involved in the basic biology of cancer (10,11). Chronic tumor cell hypoxia increases genomic instability and heterogeneity and selects for tumor cells that survive severe microenvironmental stresses (12).
The regulation of HIF-1 signaling is a rather complex process. Suppression of HIF-1α degradation has not only been observed as a result of intracellular oxygen depletion but also due to environmental stresses like extracellular acidosis (13). Most importantly, growth factors like insulin-like growth factor receptor, epidermal growth factor receptor, human epidermal growth factor receptor 2, and others can also stimulate HIF-1 signaling (14), indicating that the regulation of the HIF-1 oxygen sensing system is more complex than previously appreciated.
Hypoxia in Cardiovascular Disease
The oxidative phosphorylation of adenosine diphosphate to adenosine triphosphate is the main source of energy needed to provide sustained contractility of the heart muscle. Because of that, ischemic conditions (thus inadequate oxygen supply) are likely to cause hypoxia of variable degrees. However, under certain pathophysiological conditions such as infections, hypoxia may occur in the heart muscle even if perfusion is well maintained. One important consequence of coronary artery disease, left ventricular dysfunction, can result from acute myocardial ischemia or myocardial infarction. In addition, transient postischemic “stunned” myocardium and chronic but potentially reversible ischemic “hibernating” myocardium are also associated with left ventricular dysfunctions. It has been shown that hibernating myocardium is characterized by an up-regulation of genes and corresponding proteins involved in antiapoptosis, cell growth, angiogenesis, and cytoprotection, including the activation of the HIF-1 cascade (15). Since hibernating myocardium can be significantly improved by successful revascularization, the identification of this condition is of great clinical importance.
Currently, accessible noninvasive imaging approaches for the detection of myocardial ischemia are based on the recognition of flow impairment or the identification of a mismatch between flow and regional myocardial metabolism. All existing approaches are indirect methods assessing regional myocardial ischemia and are affected by sympathetic activation and substrate availability. The direct visualization of myocardial tissue hypoxia has great potential. It has been speculated that the assessment of tissue oxygenation
using hypoxia tracers may potentially be the best indicator of the balance between blood flow and oxygen consumption (16). Consequently, hypoxia tracers have been suggested to identify dysfunctional chronically ischemic but viable hibernating myocardium.
using hypoxia tracers may potentially be the best indicator of the balance between blood flow and oxygen consumption (16). Consequently, hypoxia tracers have been suggested to identify dysfunctional chronically ischemic but viable hibernating myocardium.
Hypoxia in Oncology
Tumor hypoxia is a common, if not characteristic, feature of malignant tumors. It is related but not exclusively determined by a less ordered, often chaotic, and leaky vasculature. The structure and function of the tumor microcirculation is often disturbed and results in a deterioration of the diffusion geometry. Tumor-associated anemia further aggravates tumor tissue hypoxia. The existence of tumor hypoxia had long been suspected by histological observation of necrosis and disordered vasculature and was confirmed in animals and humans by microelectrode and bioreductive drug measurements. Tumor hypoxia has the well-known effect of decreasing the sensitivity of hypoxic cells to ionizing radiation. It has also been identified as a major adverse prognostic factor for tumor progression and for resistance to anticancer treatment (17,18,19).
Clinically relevant tumor tissue hypoxia is generally considered to be present at an oxygen partial pressure in tissue (tpO2) of less than 8 to 10 mm Hg (12,20), while severely hypoxic tissue usually displays tpO2 values below 3 to 5 mm Hg. Tumor tissue hypoxia plays an important role in radiation treatment because the extent of DNA damage following exposure to indirectly ionizing radiation is largely dependent on oxygen. Severely hypoxic cells require two to three times higher radiation dose compared to well-oxygenated cells to produce an equivalent amount of cell kill following indirectly ionizing radiation or low linear energy transfer radiation (17). The difference in radiosensitivity between hypoxic and normoxic cells has been called oxygen enhancement ratio. Oxygen is believed to prolong the lifetime of the short-lived free radicals produced by the interaction of x-rays and cellular water. In the absence of intracellular oxygen, free radicals formed by ionizing radiation are able to recombine without causing the expected cellular damage (21). Besides causing radioresistance, tumor hypoxia interferes with many chemotherapy regimes that require sufficient amounts of intracellular oxygen for the desired cytotoxic activities (22). The inefficient microvasculature of hypoxic tumors hampers sufficient drug delivery (17). Sustained hypoxia may also reduce tumor sensitivity by indirect mechanisms that include proteomic and genomic changes, the secretion of hypoxic stress proteins, and the loss of apoptotic potential (23,24,25). Fig. 8.24.1 summarizes the current knowledge of mechanisms for hypoxia-related treatment resistance and their deleterious effects on tumor aggressiveness and metastatic potential.
Challenges of Hypoxia Measurements
Many approaches to measure tissue hypoxia have been proposed. Most of the clinical experience has been obtained with polarographic oxygen-electrode systems. Although the measurements are quantitative, the results were generally reported as the fractional percentage of measurements below a certain cutoff tpO2 value. Due to the heterogeneous distribution of tpO2 values, results were generally reported as the “hypoxic tumor fraction,” which was found to be a more robust parameter derived from such polarographic methods when compared to the average tpO2 in tissue.
In the past decade, a large body of evidence was derived from oxygen electrode measurements. Studies have subsequently shown that pretreatment oxygenation can predict outcome of treatment in several solid tumors including head and neck cancer (26,27), lung
cancer, cervical cancer (18), and sarcomas (28). However, oxygen electrode measurements are rarely used routinely in human malignancies, mainly because they are invasive, limited to readily accessible tumor sites, and technically demanding (29). The exact localization of the probe’s tip within the tumor volume is difficult, and oxygen electrode systems are unable to determine the tumor’s oxygenation distribution on a truly regional basis, which is a necessary precondition for individually adapted therapeutic approaches. A noninvasive identification and quantification of regional tumor tissue hypoxia is, therefore, nearly a necessity for effective treatment selection, individual treatment planning, and treatment monitoring in oncology.
cancer, cervical cancer (18), and sarcomas (28). However, oxygen electrode measurements are rarely used routinely in human malignancies, mainly because they are invasive, limited to readily accessible tumor sites, and technically demanding (29). The exact localization of the probe’s tip within the tumor volume is difficult, and oxygen electrode systems are unable to determine the tumor’s oxygenation distribution on a truly regional basis, which is a necessary precondition for individually adapted therapeutic approaches. A noninvasive identification and quantification of regional tumor tissue hypoxia is, therefore, nearly a necessity for effective treatment selection, individual treatment planning, and treatment monitoring in oncology.
PET Radiopharmaceuticals for Hypoxia Imaging and Preclinical Testing
Nitroimidazole Compounds
The discovery of azomycin (2-nitroimidazole) (30) and its synthesis in 1965 (31) started decades of research in hypoxic cell radiosensitization and was the basis for the development of a whole group of nitroimidazole tracers for single-photon emission computed tomography and positron emission tomography (PET) imaging. Chapman (32) suggested that nitroimidazoles should be useful for imaging of oxygen-deprived cells due to their radiosensitization capabilities and potential covalent binding to hypoxic cells. Intracellular accumulation is related to radical formation following reduction by ubiquitous nitroreductases. Under hypoxic conditions, reduction of these molecules involves a series of one electron steps (33). Products of this reduction are believed to covalently bind to intracellular macromolecules such as DNA, RNA, and proteins, which prevents back-diffusion across the cell membrane, causing the hypoxia-dependent accumulation of compound metabolites. Conversely under well-oxygenated conditions, the nitro radical anion of these compounds are reoxidized, facilitating back-diffusion and contributing to clearance of radioactivity from tissue (34). However, even under hypoxic conditions, some diffusible compounds are formed indicating complex biokinetics.
Fluorine-18 labeled fluoromisonidazole (1-(2-nitroimidazolyl)-2-hydroxy-3-fluoropropane) ([18F]-FMISO) was the first nitroimidazole PET tracer described and synthesized by Jerabek et al. (35) and Grierson et al. (36). The evaluation of this tracer was first performed using [3H]-FMISO, demonstrating that the FMISO uptake was dependent on the cellular oxygenation in rat myocytes (37) and tumor cell spheroids (38). Studies performed in several rodent tumor models using [3H]-FMISO revealed tumor-to-blood ratios suitable for in vivo imaging (39,40). The hypoxia-specific uptake mechanism of [18F]-FMISO was validated in an occlusion model and demonstrated tracer accumulation at radiobiologically relevant tpO2 levels (41,42). The radiopharmaceutical [18F]-FMISO is stable and robust and is currently the single most commonly used hypoxia PET tracer (43). However, its biokinetics suffer from relatively high lipophilicity (octanol/water partition coefficient of log P = 2.6), which results in protracted in vivo accumulation in hypoxic tissues and slow plasma clearance, resulting in relatively low target-to-background ratios. Imaging with nitroimidazole compounds is, therefore, generally performed at later time points (2 to 4 hours postinjection) when sufficient amounts of radioactivity have been cleared from plasma and normoxic tissues.
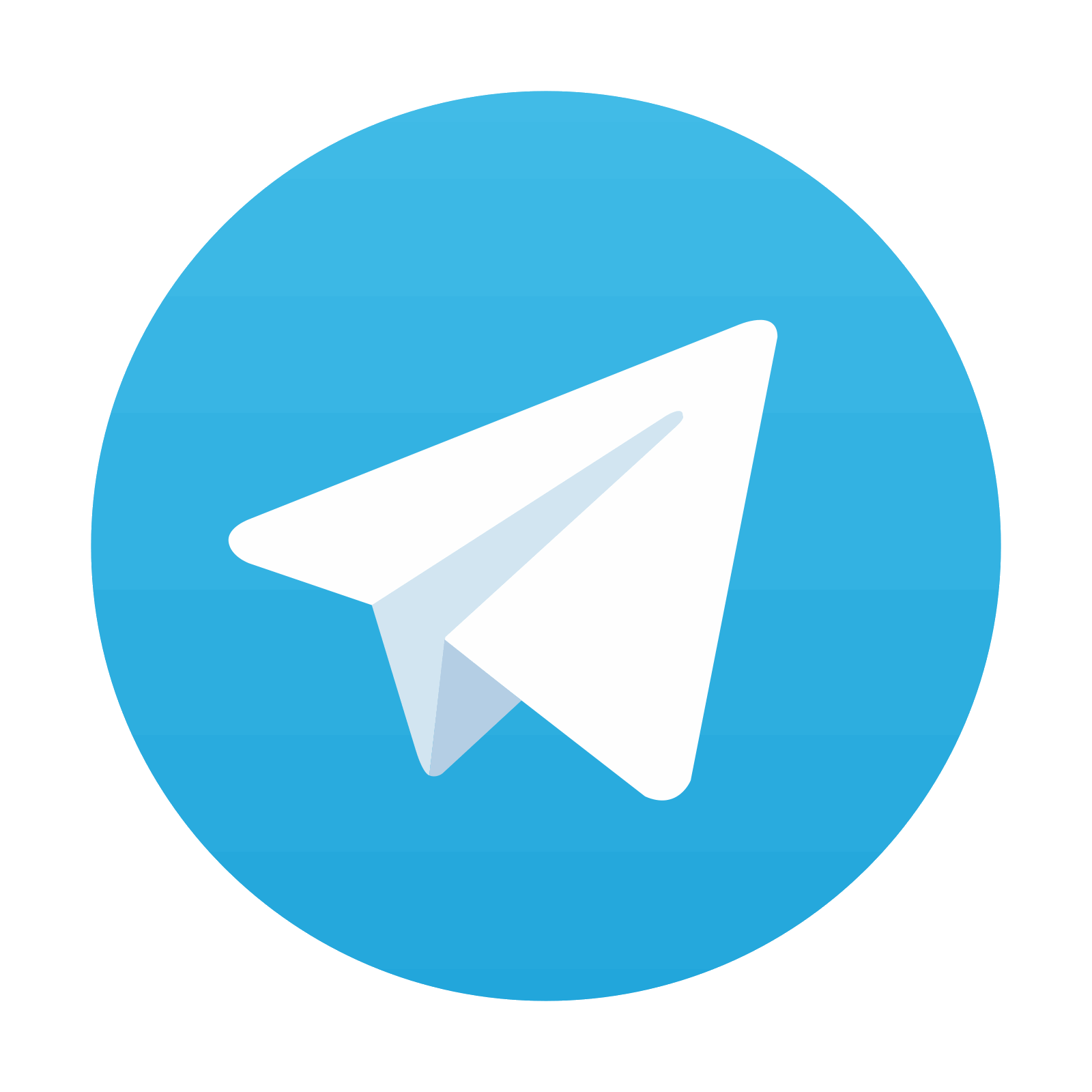
Stay updated, free articles. Join our Telegram channel
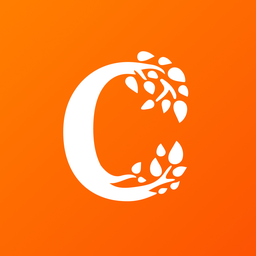
Full access? Get Clinical Tree
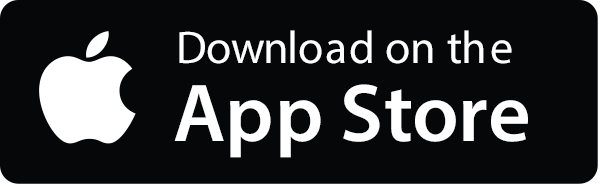
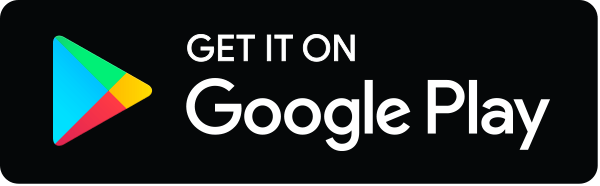