Fig. 1
Intensity-modulated radiotherapy (IMRT). The IMRT treatment plan for re-irradiation based on the FET uptake of the patient imaged in this figure. This plan of 39 Gy in 13 fractions consists of two arcs of gantry movement. One arc composed of more than 150 fields, indicated by the yellow circle. The concave and convex dose distribution is best visualized in the blue 50 % isodose line
A nonuniform dose distribution can be intentionally prescribed in dose painting to target tumor areas that are markedly radiation resistant. These areas can be visualized on PET (e.g., hypoxia PET, proliferation PET, etc.) or functional MRI. Alternatively, a more conformal dosage can be obtained by a simultaneous integrated boost in which all target volumes such as viable tumor and residual tumor plus margins are treated concurrently (Piroth et al. 2012). These complex plans are evaluated on the basis of dose-volume tables and histograms that show minimum, mean, and maximum dose to a given structure. Presently there is no proven benefit to delivering doses beyond 60 Gy with IMRT in glioblastoma patients (Chan et al. 2002).
2.4 Stereotactic Radiosurgery (SRS) and Radiotherapy
Stereotactic radiosurgery (SRS) and radiotherapy are delivered using a linear accelerator or Gamma Knife with cobalt-60. Stereotactic radiosurgery is performed in a single high-dose fraction to small (<4 cm) targets, whereas fractionated stereotactic radiosurgery is delivered in several fractions. Stereotactic radiotherapy can also be delivered over multiple fractions.
The Gamma Knife contains a helmet with circular apertures ranging from 4 to 18 mm that collimates cobalt-60 rays onto a single target point. In a linear accelerator, circular collimators ranging from 4 to 40 mm diameter generate a circular pencil beam. The treatment is delivered using multiple non-coplanar arcs that intersect at a single point. The ideal target is spherical in shape. Irregularly formed lesions are treated using multiple circular collimators or collimator helmets placed on different nearby target points to minimize the exposure of normal brain. RTOG study 90–05 recommended the maximum tolerated dose of single fraction SRS to be 24 Gy to a target ≤20 mm, 18 Gy to a target of 21–30 mm, and 15 Gy to a target of 31–40 mm (Shaw et al. 2000).
LINAC radiosurgery is performed by linear accelerators incorporating improved guiding techniques and methods like micromultileaf collimators or intensity modulation for improved accuracy.
CyberKnife is a LINAC-based commercially available system mounted on an industrial robot. The robotic arm can be manipulated in six axes. The LINAC system provides energy of 6 MeV and uses circular collimators. Two orthogonal X-ray apparatus are used for target tracking leading to frameless positioning of the patient. According to the manufacturer, an accuracy of 0.2 mm can be achieved.
RTOG 93–05 trail was unable to demonstrate a benefit from adjuvant SRS in a phase III trial, in which 203 patients were randomly assigned to stereotactic radiosurgery followed by involved field RT plus carmustine (BCNU) to immediate involved field RT plus carmustine. Median survival was similar on both arms of the study (13.6 and 13.5 months, respectively), as was survival at 2 and 3 years (21 versus 19 % and 9 versus 13 %, respectively) (Souhami et al. 2004). Conversely, stereotactic radiosurgery has also been used to boost fractionated RT for the treatment of newly diagnosed GBM following either biopsy or resection. Results from observational studies have been mixed and are difficult to interpret due to patient selection bias.
2.5 Interstitial Brachytherapy
For interstitial brachytherapy, radioisotope seeds are placed intraoperatively within the tumor or resection cavity. Iodine-125, a low-dose rate irradiator, is commonly used. The high-dose isotope iridium-192 has also been tested for selected patients. Although interstitial brachytherapy is frequently used in treatment of other disease entities, such as prostate cancer, its role is limited in the treatment of gliomas.
Brachytherapy enables the delivery of a large radiation dose to the tumor volume, with rapid falloff in surrounding tissues. Despite these theoretical dosimetric and radiobiological advantages, randomized clinical trials have shown marginal or negligible benefit in the treatment of malignant gliomas. In the largest study, 299 patients with malignant gliomas were randomly assigned postoperatively to IFRT plus carmustine with or without interstitial brachytherapy. The difference in median survival with the addition of brachytherapy was not statistically significant, 68 versus 59 weeks without brachytherapy (Selker et al. 2002).
2.6 Dose Prescription
An RT dose of 50–60 Gy has been shown to maximize postoperative survival, independent of the extent of resection (Coffey et al. 1998). Dose escalation above 60 Gy did not improve survival and was associated with severe white matter changes which correlate with the total dose of cranial irradiation (Piroth et al. 2012; Corn et al. 1994; Tsien et al. 2009).
Typically, WHO grade III gliomas are treated with a dose of 59.4 Gy in 1.8 Gy fractions versus 60 Gy in 2 Gy fractions for grade IV. This 10 % dose reduction per fraction is postulated to reduce the extent of normal tissue complications in patients with protracted survival, but there is no data comparing these regimens.
2.7 Particle Radiation Therapy
Particles used for radiation therapy include atomic particles such as electrons, neutrons, and protons (hydrogen), as well as nuclei of atoms such as helium, carbon, and neon. Heavy ion particles such as helium and neon directly damage cellular targets, rather than working through a free radical intermediary that is oxygen dependent, such as with electrons and photons. This may enhance the efficacy of treatment in the hypoxic conditions present in certain tumors such as malignant gliomas. In addition, these heavy ion particles release energy at a certain depth, known as a Bragg peak, which allows the dose to be precisely aimed within the target tissue and provide an increased relative biological effectiveness. They have been used alone and as a boost to conventional photon EBRT (Combs et al. 2010).
Prospective randomized phase III trials comparing protons or carbon ions with precision photon RT have never been conducted. There are limited data on the usage of proton beam RT in GBMs. A study of 15 patients with GBM treated with neon ion irradiation showed median survival of 13–14 months (Castro et al. 1997). In one series of 23 patients, proton RT resulted in a median survival of 20 months following surgery (Fitzek et al. 1999). In the later study a dose equivalent to 90 Gy was prescribed. The authors reported a high rate of tissue necrosis causing progressive neurological symptoms and need for surgical intervention.
Whereas the highly conformal radiation delivery with the use of protons can permit dose escalation, its potential application in treating GBM may be better suited to simply limiting RT-related side effects. Radiation therapy using protons can limit RT-related side effects due to its conformal dose delivery. However, a convincing benefit from dose escalation was not demonstrated so far. These data do not appear to be better than can be achieved with standard photon RT.
Indications for heavy particle include skull base chordomas and chondrosarcomas (Schulz-Ertner and Tsujii 2007). In a retrospective analysis of nonrandomized treatment groups, chordoma patients treated with protons had a significantly higher local control probability in comparison to patients treated with photons (Colli and Al-Mefty 2001).
3 Role of Imaging and Treatment Planning
In radiation oncology, imaging is used for clinical staging and treatment planning. Following a course of treatment, imaging is performed at regular intervals to assess for recurrence or, in case of long-term survivors, for second primary malignancies. Radiation oncologists must be familiar with imaging modalities and understand the accuracy and limitations of each. Considering the abovementioned improvements and changes of radiation therapy, the role of imaging becomes more and more important in treatment planning.
An important part of the radiation therapy is the delineation of the gross tumor volume (GTV), clinical target volume (CTV), and planning target volume (PTV). The macroscopic apparent tumor volume is referred to as GTV. The CTV encompasses suspected tumor invasion to the adjusted tissue, lymph vessels, or the draining lymph node stations, which cannot be identified by imaging. Radiation oncologists need expertise in anatomy and knowledge of the different malignancies in order to generate a rational and individual CTV. For example, glioblastoma spread along the white matter does not infiltrate the dura. The planning target volume (PTV) takes into account the accuracy of administration of the radiation therapy. A safety margin is added to the CTV in all directions depending on the accuracy of the treatment facility, the radiotherapy technique, the reproducibility of the daily patient positioning, or the patient movement during treatment.
Standard morphological imaging methods like CT (computed tomography) and MRI (magnetic resonance imaging) enhanced with contrast agents are employed.
In the treatment of GBM, the RT dose is usually delivered to the tumor or resection cavity plus a margin of apparently normal brain tissue. The EORTC guidelines (European Organization for Research and Treatment of Cancer) recommend a margin of 2 cm. The current guidelines of RTOG recommend a 2 cm margin around the resection cavity and the postoperative edema. In a recent study, patterns of failure were similar between the different treatment plans; however the median volume percent of brain irradiated to high doses was significantly smaller for EORTC plans than for RTOG plans (Minniti et al. 2010).
Margins from CTV to PTV became smaller over the years because of improvement of patient positioning using stereotactic cranial masks (STX) or STX whole body mat. Image guidance during RT not only controls the positioning of the patient but also the positioning or filling of inner organs. For this purpose, X-rays, kV imaging or MV imaging, and even cone beam CT are integrated in the linear accelerator.
Advanced imaging modalities for the RT treatment planning require sophisticated software tools to co-register multiple imaging scans. The automatic segmentation of structures can be done according to density information like Hounsfield units or by generating iso-contours by defining thresholds. Some programs can also generate structures like organs at risk from a stored database.
3.1 Computed Tomography (CT)
CT plays a primary role in RT treatment planning. Compared with conventional simulation, CT-based planning allows for more accurate target delineation, tighter margins, and less normal tissue irradiation. CT dose calculation is based on tissue density (Hounsfield units).
3.2 Magnetic Resonance Imaging (MRI)
Due to excellent soft tissue contrast and ability to image directly in multiple planes, MRI is the preferred imaging modality for intracranial and spinal tumors. Using modern software, MRI is co-registered to the planning CT.
The contrast enhancement on MRI represents the breakdown of the blood–brain barrier (BBB) (Conventional MR imaging). A disruption of the BBB can also occur from recent operations or radiation therapy (Taal et al. 2008; Clarke and Chang 2009; Wen et al. 2010). Thereby, it is not tumor specific. Additionally, there are sometimes large tumor parts where the BBB is not yet affected and that show no secondary tumor features like enhancement, cerebral edema, and/or compression of other brain structures. These tumor parts as well as non-enhancing low-grade gliomas are only seen as a hyperintense signal on FLAIR or T2 sequences (Conventional MR imaging). In these cases, the contrast enhancement in T1-weighted MRI sequences underestimates the tumor mass, and consequently, these parts are insufficiently treated.
In addition, as bevacizumab reverses the breakdown of the BBB, it leads to decrease of the contrast enhancement on MRI, pseudo-response, and alteration in tumor behavior. There is evidence that bevacizumab may alter the recurrence pattern of malignant gliomas by suppressing enhancing tumor recurrence more effectively than it suppresses nonenhancing, infiltrative tumor growth (Norden et al. 2008).
Consequently, as mentioned in Conventional MR imaging, certain treatment-related changes after surgery, radiation, and/or chemotherapy, known as pseudo-progression and pseudo-response, cannot be differentiated from tumor tissue (Clarke and Chang 2009; Wen et al. 2010; Brandsma and van den Bent 2009). Because it is self-limiting, it is necessary to separate this phenomenon from radionecrosis, which is a late and progressive radiation injury. It can develop months or even years after the treatment (Giglio and Gilbert 2003).
3.3 Positron Emission Tomography (PET)
Imaging methods like positron emission tomography (PET) and single-photon emission computed tomography (SPECT) detect metabolic activity of tumor tissue.
As described in Chap. 7, current diagnostic efforts focus on the use of radiolabeled amino acids (AA) as tracers for brain tumors. Increased AA uptake in gliomas is related to an overexpression of amino acid transporters in the cell membrane. It has been demonstrated that AA uptake in tumor tissue is almost entirely mediated by type L-AA carriers (Langen et al. 2000).
FET and MET were shown to be equally sensitive and specific in clinical practice (Weber et al. 2000; Grosu et al. 2011).
PET and MRI scans of gliomas show considerable differences in tumor volume and position (Fig. 2). In 39 resected GBM patients 11C-MET uptake extended beyond the tumor identified by magnetic resonance imaging in 74 % (Grosu et al. 2005a). In a study of 41 glioma patients, integrating FET uptake into the delineation of GTVs yielded significantly larger volumes in high-grade patients. The congruence of MRI and FET signals was poor with mean uniformity indices of 0.39. MRI-based PTVs missed 17 % of FET PET-/CT-based GTVs (Rieken et al. 2013). Thus, the choice of imaging technique can affect the success of the radiotherapy.
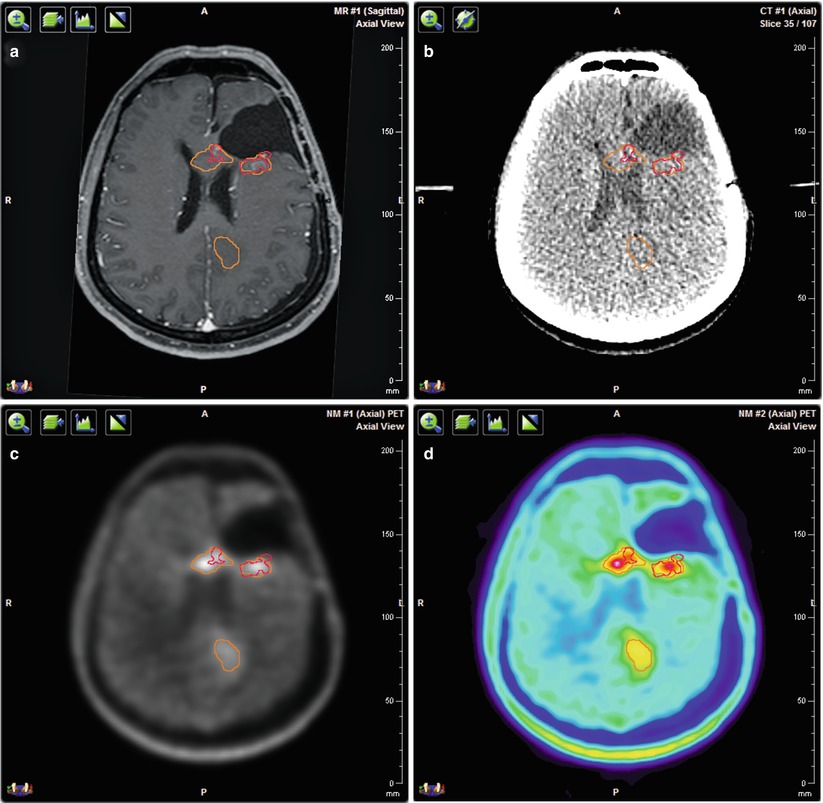
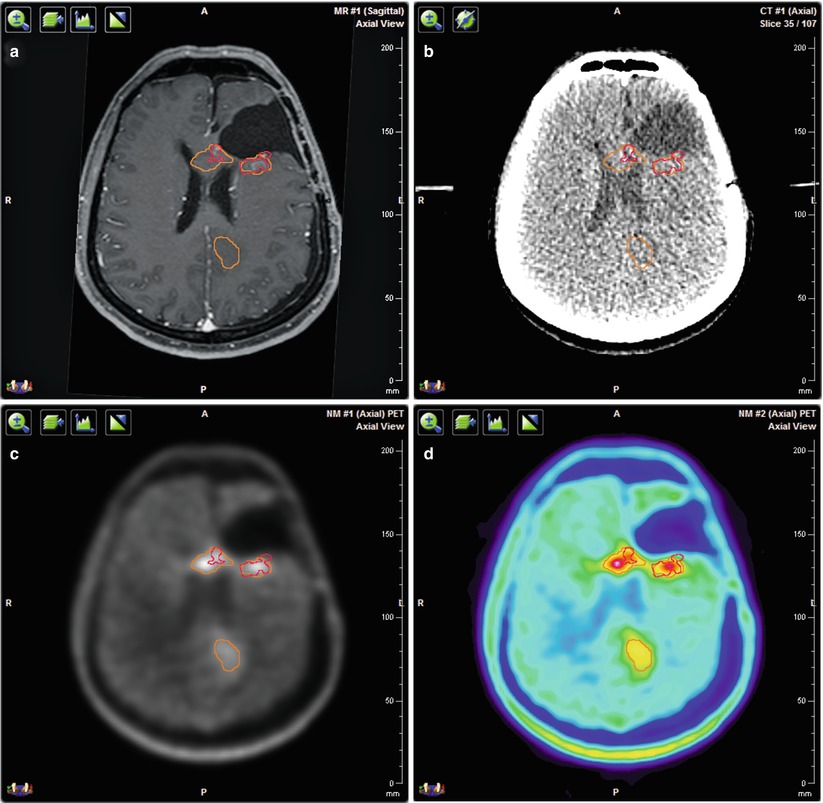
Fig. 2
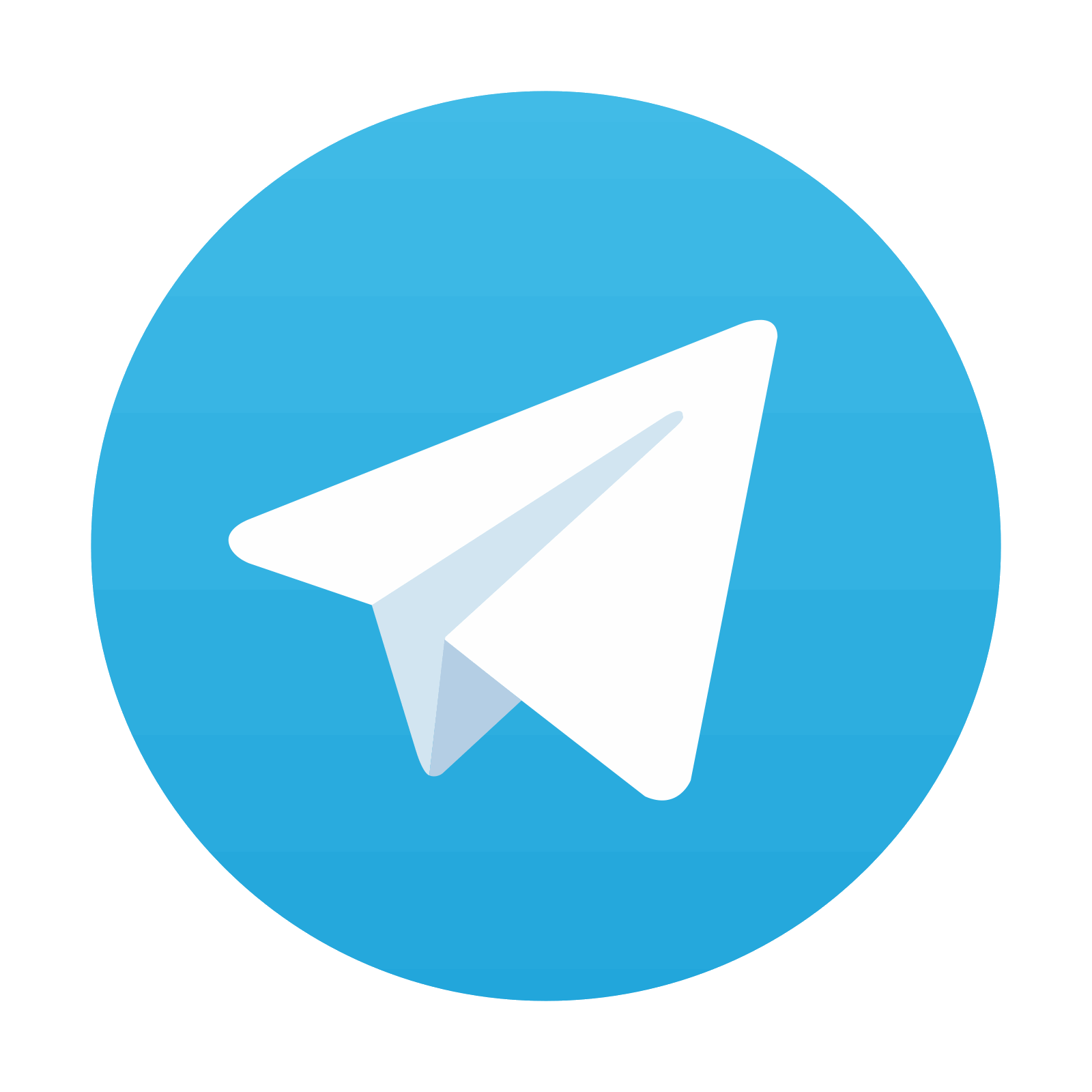
Multimodal imaging for target volume delineation in a patient with recurrent glioblastoma. (a) Three-dimensional T1-weighted contrast enhanced MRI. (b) Planning CT. (c) 18F-FET PET/CT with CT-based attenuation correction in gray scale. (d) 18F-FET PET/CT with CT-based attenuation correction in rainbow scale. All images are co-registered with the planning CT. The red contour corresponds to the contrast enhancement on MRI and the orange contour to the FET uptake on PET. The volume and position of the detected tumor vary depending on the imaging method
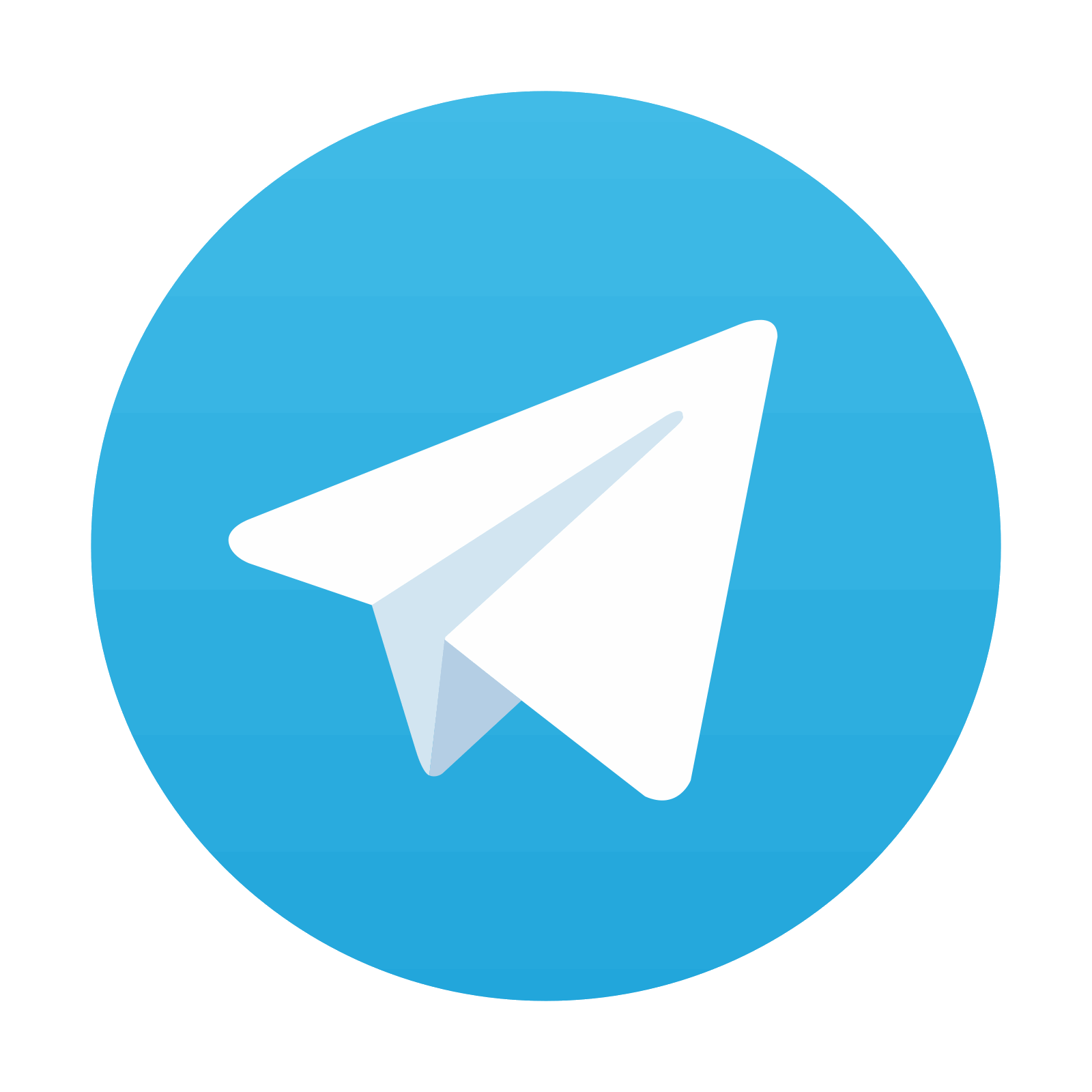
Stay updated, free articles. Join our Telegram channel
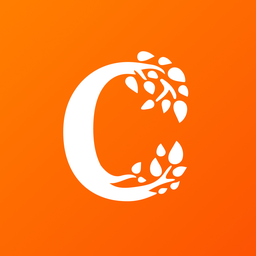
Full access? Get Clinical Tree
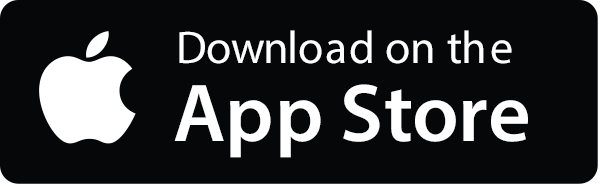
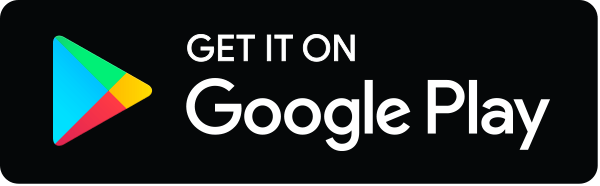
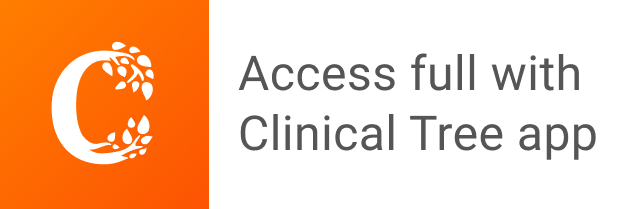