Chapter 6 Imaging with radionuclides
Introduction
This medical imaging modality is classified by the radionuclide gamma ray emission which provides the energy for the ordered transfer from the object to the image. Radionuclide imaging is primarily concerned with the function of organs and tissues. The other modalities, x-ray, magnetic resonance imaging (MRI) and ultrasound, primarily convey structural or anatomical information, though the distinction between structural and functional imaging is not absolute. The detected signal is often processed in some way before being displayed. Multimodality combinations of images, as in positron emission tomography and x-ray computed tomography (PET-CT), can provide an especially powerful tool for discriminating between normal and malignant tissues [1].
Overview of the radionuclide imaging process
Nuclear medicine departments routinely perform diagnostic imaging and non-imaging procedures and, in many hospitals, undertake radionuclide radiotherapy treatments. However, in this chapter, only radionuclide imaging will be described and therapeutic applications are dealt with in Chapter 7. A radionuclide is an unstable isotope of an element that will spontaneously ‘decay’ by the emission of particles and/or electromagnetic radiation. The three most common emissions from radionuclides are alpha and beta particles and gamma rays. There are two types of beta particle, beta minus (β−) and beta plus (β+), they are both electrons with a negative and positive charge, respectively. A beta plus is referred to as a positron. A gamma ray is a form of electromagnetic radiation and is the only one with sufficient penetrating characteristics to enable it to be detected externally if originating from within the body. Most use is made of a relatively limited number of radionuclides that emit only gamma rays. However, there is a growing use of radionuclides that decay by emitting a positron; this particle is not penetrating but interacts with an electron, both annihilating to form two photons travelling in opposite directions. These photons are penetrating and can be detected as they leave the body. A radionuclide is attached, or labelled, to a pharmaceutical, forming a radiopharmaceutical. This is introduced into the body, most commonly by intravenous injection but also by ingestion and inhalation, and has a known biodistribution in the body. The distribution of the radiopharmaceutical is related to the physiology or function of an organ, tissue or tissue type; this is in contrast to x-ray, MR or ultrasound imaging which, to a large extent, images structure.
The most up-to-date gamma cameras and all PET cameras are now manufactured with an x-ray CT imaging device incorporated into the gantry. In both cases, the primary purpose of this ancillary facility is to allow the use of the structural CT (transmission) images to be converted into an attenuation map [2]. This map is used to correct the functional nuclear medicine (emission) images for variation in body tissue thickness and density. Having the CT imaging incorporated into the cameras allows the emission and transmission images to be acquired sequentially in the same patient imaging session. Assuming there is no patient movement, the data derived from the transmission image can be accurately applied to the emission images. This process is applicable to SPECT images acquired on gamma cameras and all images acquired on PET cameras. Consequently, imaging techniques associated with these devices are known as SPECT/CT and PET/CT. Initially, the CT units were not of a standard comparable to the most up-to-date helical multislice devices and the structural images obtained were not of a diagnostic quality. However, gamma cameras and PET scanners can now be purchased with high specification CT units attached and the registration or fusion of high spatial resolution structural images with the sensitive but poor resolution function images results in an imaging device that is extremely effective at identifying and localizing pathology.
Gamma cameras
Gamma cameras (Figure 6.1) are imaging devices used to detect and record the distribution of a radiopharmaceutical within the body. Gamma rays are detected by a large area crystal of sodium iodide doped with trace quantities of thallium (NaI(Tl)), the crystal structure has the property of absorbing the gamma radiation and re-emitting photons of visible light; the crystal is referred to as a scintillator. The crystal dimensions vary but are typically 50 cm × 40 cm with a thickness of about 1 cm. Smaller detector systems are available for specialized purposes, such as cardiac or brain imaging. A schematic of a gamma camera detector head is shown in Figure 6.2. Further information regarding the design of gamma cameras may be found in Cherry et al [3].
The process of recording a single gamma ray event by the camera is as follows:
• a γ-ray emitted from the body and travelling towards the detector, in a direction approximately parallel to collimator holes (parallel hole collimator), will be able to pass through and interact with the NaI(Tl) scintillator crystal
• the γ-ray will be absorbed by the crystal and the energy will be re-emitted as a plume of photons with a wavelength associated with visible light
• the light photons travel through a waveguide to the photomultiplier tube (PMT) array where they are converted into photo-electrons
• the photo-electrons are multiplied between the dynodes of the PMT, amplifying the signal
• circuitry between the PMTs produces information about the position of the detected event
• the total output from the PMT array gives information about the energy of the detected event. Energy discriminators allow photons of a specific energy, usually only unscattered photons, to contribute to the image.
Imaging techniques
Tomographic imaging
Knowledge of the 3D distribution of the radiopharmaceutical within the body allows correction for the attenuation of the emitting gamma radiation if the size and density of surrounding structures can be determined. This is achieved with the aid of a CT attenuation map. A CT acquisition represents the variation of x-ray absorption throughout the image volume, a direct relationship can be made between this and the attenuation of the emitted gamma radiation. An example of nuclear medicine images that have been attenuation corrected and fused with the CT structural images is shown in Figure evolve 6.6 . Note that the CT device in this example is a low dose unit, not in the diagnostic category.
Gamma camera performance characteristics
Detailed descriptions of the precise definitions of these parameters, factors influencing their stability, measurement methodologies and how they may be specified during equipment procurement are covered in two publications; IPEM 2003 [4] and NEMA 2007 [5]. Brief outlines are given here.
There are additional performance characteristics to take into account when performing SPECT imaging and still further with SPECT/CT. SPECT imaging requires the centre of rotation (COR) of the rotating camera heads to be within predefined limits. Failure to do so will result in artifacts on the reconstructed SPECT images. It is also important that the parameters defining the detector heads remain stable at every angle of rotation. SPECT/CT relies on the accurate registration of the SPECT and CT images. The performance of the CT is assessed as for all CT equipment, as covered by IPEM Report 91 [6]. A crucial additional assessment is the alignment of the SPECT and CT data together. Phantoms are routinely used to assess the gamma camera SPECT capability and SPECT/CT alignment.
Positron emission tomography scanners
Dedicated positron emission tomography cameras (Figure 6.3) are designed specifically for radionuclides that decay by positron emission. Scintillator crystals with high stopping power are utilized, examples are bismuth germanate (BGO) and lutetium orthosilicate (LSO). These crystals, coupled to photomultiplier tubes, are positioned in rings around a gantry. Electronic circuitry, designed to register if two crystals detect a photon within a ‘coincidence time’, usually a few nanoseconds, allow ‘true’ events to be detected. Many ‘single’ events will be detected. The size of the crystal elements depends on the make and model, but is generally 3 to 6 mm. The elements are arranged in 360° rings, with typically 15 to 30 rings consisting of the order of 10 000 individual crystal elements. The crystal size is one of the main factors in limiting the spatial resolution, commonly 4 mm (FWHM) [7]. Although systems designed for special purposes, such as brain scanning, can achieve 2 mm. The computer system is able to reconstruct the line connecting two detected events in opposite crystals as a line of response (LOR). Millions of LORs are acquired over a period of time and reconstructed to form cross-sectional images using appropriate image reconstruction software.
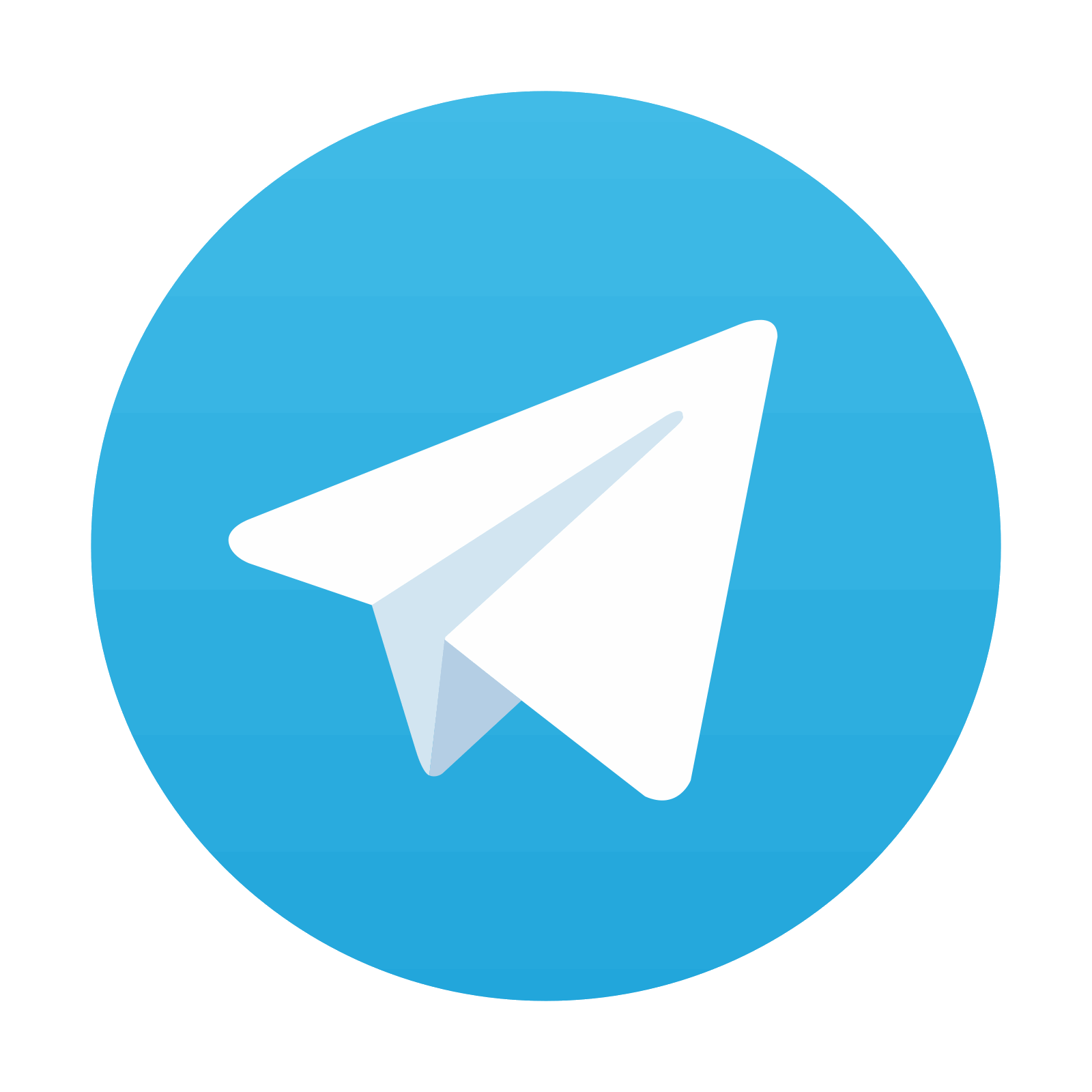
Stay updated, free articles. Join our Telegram channel
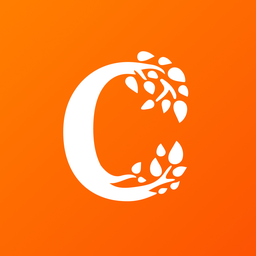
Full access? Get Clinical Tree
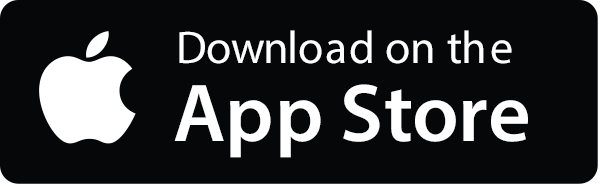
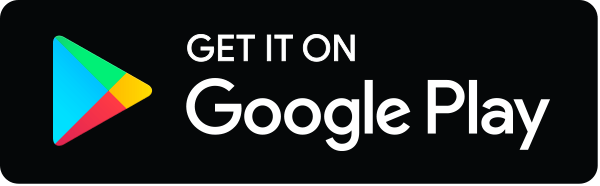