- •
Iron cardiomyopathy remains the leading cause of death in transfusional siderosis.
- •
Heart and endocrine gland iron overload occurs from chronic exposure to nontransferrin bound iron.
- •
Different organs have unique kinetics of iron uptake and clearance.
- •
Serum ferritin and liver iron are useful but insufficient markers of cardiac and endocrine risk.
- •
Cardiac magnetic resonance (CMR) can be use to quantitate iron deposition in many tissues, including the heart.
- •
CMR visualizes the safely stored iron, so patients may be presymptomatic, but relative risk of organ complications increases proportionally to the amount of stored iron.
- •
Annual measurements of liver iron, cardiac iron, and cardiac function are becoming the standard of care in transfusional siderosis.
- •
Cardiac toxicity is completely reversible in most situations with intensive chelation, although full recovery may take years.
DISEASE RESPONSIBLE FOR IRON OVERLOAD
Table 12-1 lists the most common diseases responsible for primary (nontransfusional) iron overload as well as the most common forms of secondary (transfusional) siderosis. Hemochromatosis types 1 to 4 represent hyperabsorption syndromes. Genetic defects in key iron regulatory proteins lead to loss of negative feedback of iron absorption. Primary iron ingestion, outside of acute overdosing, is generally restricted to cultures that perform fermentation in iron vessels. Some porphyrin pathway defects are associated with hepatic and extrahepatic iron accumulation. Liver disease, resulting from alcohol, hepatitis, and metabolic syndromes, often produces isolated hepatic siderosis. Cardiac iron has been well documented in hereditary hemochromatosis, particularly types 1 and 2, but is otherwise relatively uncommon in nontransfusional siderosis. Thalassemia major and sickle cell disease are among the most common genetic disorders in the world. Because genetic heterozygosity conveys a survival advantage for malaria, disease prevalence is greatest in the Middle East, Mediterranean, Africa, India, China, and the Far East. Iron overload in myelodysplastic syndromes is becoming more problematic as improved chemotherapeutic regimens lengthen life expectancy from the primary disease. Similarly, improved outcomes in a number of cancer therapies have increased the number of survivors bearing large transfusional iron stores. Cardiac iron overload is relatively common in all forms of transfusional siderosis, depending on the intensity and duration of transfusional support.
NONTRANSFUSIONAL IRON OVERLOAD | TRANSFUSIONAL IRON OVERLOAD |
---|---|
Hereditary Hemochromatosis | Thalassemia major |
Type 1: HFE | Sickle cell disease |
Type 2: Hemojuvelin, Hepcidin | Myelodysplastic syndromes |
Type 3: TFR2 | Blackfan-Diamond syndrome |
Type 4: Ferroportin | Congenital dyserythropoietic anemia |
Iron ingestion | Pure red cell aplasia |
Heme metabolism disorders | Iatrogenic marrow suppression |
Liver disease |
ORGANS AFFECTED BY IRON OVERLOAD
The anterior pituitary, particularly gonadotrophic cells, appear to be the most sensitive organs to iron deposition ( Table 12-2 ). However, iron deposition occurs in all endocrine organs with variable clinical penetrance. Liver damage includes fibrosis, cirrhosis, and hepatocellular carcinoma, although clinical manifestations are generally delayed compared with the other disorders. Cardiac iron deposition occurs in approximately two thirds of adults with thalassemia major and remains the leading cause of death. Prevalence of iron-mediated complications is less well characterized in other forms of transfusional siderosis. Early diagnosis has greatly reduced organ complications in nontransfusional siderosis.
ORGAN | CONSEQUENCE | AGE OF ONSET | POPULATION PREVALENCE |
---|---|---|---|
Pituitary | Hypogonadism, growth failure | Childhood | 35%-55% |
Pancreas | Diabetes | Adulthood | 6.4%-10% |
Thyroid | Hypothyroidism | Adulthood | 9%-10.9% |
Parathyroid | Parathyroidism | Adulthood | unknown |
Adrenal | Adrenal insufficiency | Adulthood | unknown |
Liver | Fibrosis, cirrhosis, malignancy | Adulthood | 14% |
Heart | Arrhythmia, heart failure | Adolescence | ≈10% |
Bone | Arthritis, osteoporosis | Adulthood | Variable |
IRON ADSORPTION AND TRANSPORT
Inorganic iron (Fe 2+ ) enters the body via dimethyl trans-ferase 1 (DMT1) in the enterocyte with assistance of the colocalized reductase duodenal cytochrome B (DCytB). A separate transporter, heme carrier protein 1 (HCP1), is responsible for transporting heme moieties resulting from breakdown of animal products. Within the enterocyte, free iron interacts with the iron storage protein ferritin as well as iron response elements that regulate iron-trafficking gene expression. Iron export through the apical membrane occurs through a transmembrane channel known as ferroportin and its colocalized reductase, haephestin. Figure 12-1 illustrates the paths of iron adsorption and transport.
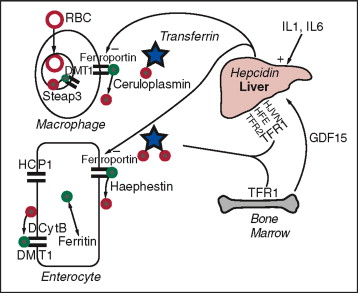
The second major source of intravascular iron is through red blood cell phagocytosis in hepatic and splenic macrophages. Free iron is pumped out of intracellular lysosomes via DMT1 (assisted by a reductase, STEAP1) and exported across the plasma membrane via ferroportin. A circulating reductase, ceruloplasmin, assists in oxidizing and loading the exported iron onto transferrin.
Transferrin shuttles iron to the bone marrow for subsequent red cell synthesis and to the liver for storage. In the liver, transferrin binds to a transferrin receptor complex consisting of transferrin receptor 1 (TFR1), transferrin receptor 2 (TFR2), hemojuvelin (HJV), and hfe protein. Upon binding, transferrin-TFR1 complexes are internalized into lysosomes and the TFR1 is recycled. The latter constituents of the TFR complex represent an iron-sensing element that regulates production of the hormone hepcidin. When transferrin saturation is high, hepcidin levels rise. Circulating hepcidin causes internalization of ferroportin in enterocytes and macrophages, lowering transferrin saturation and free iron levels.
In addition to transferrin saturation, hepcidin is positively regulated by the inflammatory cytokines interleukin 1 and interleukin 6, leading to low circulating iron levels during infections or other times of stress. This represents the primary mechanism of the anemia of chronic disease. Hepcidin is negatively regulated by GDF15, an erythroblast-derived protein produced during severe anemia and during pregnancy. This facilitates iron absorption and transport to the bone marrow. The iron hyperabsorption observed in thalassemia intermedia syndromes is produced through this mechanism.
IRON STORES AND FLUXES
Normal iron uptake from the gut is around 1 mg/day and balances iron losses through sloughing of intestinal endothelia ( Figure 12-2 ). Most of the iron (1800 mg) is consumed in the production of red blood cells in the marrow (lumped together as the “Erythron”). Muscle holds approximately 300 mg, mostly in the form of myoglobin. Liver has a dynamic repository of approximately 1000 mg. With such small iron input, there must be efficient iron recycling of senescent red blood cells by reticuloendothelial macrophages. Under normal conditions transferrin is only 30% to 40% saturated and there is no nontransferrin bound iron (NTBI). Extrahepatic organs do not take up significant amounts of transferrin bound iron. Chronic transfusion therapy adds approximately 0.3 to 0.5 mg/kg/day of iron (18 to 30 times the normal flux) into the erythron, overwhelming spontaneous elimination mechanisms. Transferrin carrying capacity saturates, producing toxic NTBI. NTBI is taken up readily by extrahepatic tissues, causing oxidative damage. NTBI also readily enters the liver and is significantly more toxic to the liver than transferrin bound iron, stimulating fibrosis and inflammation.
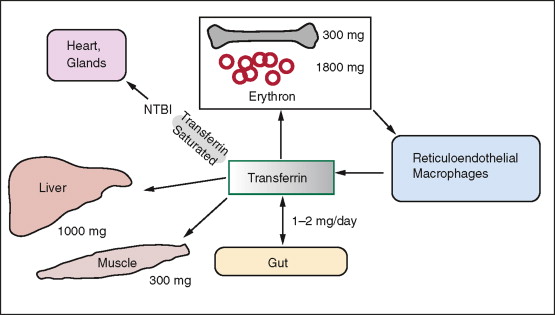
IRON ENTRY AND TOXICITY IN THE HEART
Iron enters the myocyte through divalent channels; both L-type voltage dependent channels and DMT1 have been implicated in different animal models ( Figure 12-3 ). Transferrin-mediated uptake appears to be several orders of magnitude less important. Within the myocyte, ferritin quickly binds free iron and begins shuttling it to lysosomes for degradation and long-term storage. Iron stored with the lysosome is CMR-visible and forms the basis of noninvasive monitoring. If ferritin capacity is sufficient to maintain low labile iron stores, the heart has normal function despite CMR-detectable iron. However, when this capacity is overwhelmed, free-iron interacts with cardiac iron channels as well as catalyzing oxidative stress. Lipid peroxidation weakens lysosomal membranes, leading to rupture and further iron release in a catastrophic cascade. Iron impairs mitochondrial electron transduction and energy production. Ferrous iron noncompetitively inhibits the ryanodine calcium release channel, causing profound systolic dysfunction. Iron also compromises conduction through the fast sodium and delayed rectifier potassium channels, blunted conduction velocity and delaying repolarization. Last labile iron interacts with iron response elements to modify genetic transcription, promoting release of fibrinogenic factors.
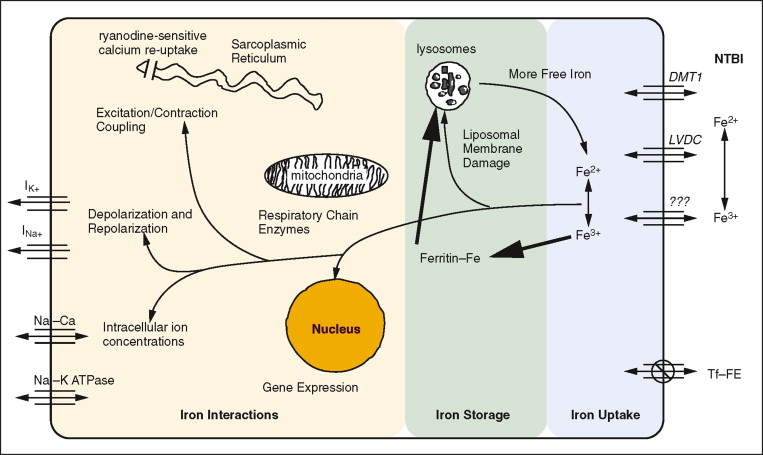
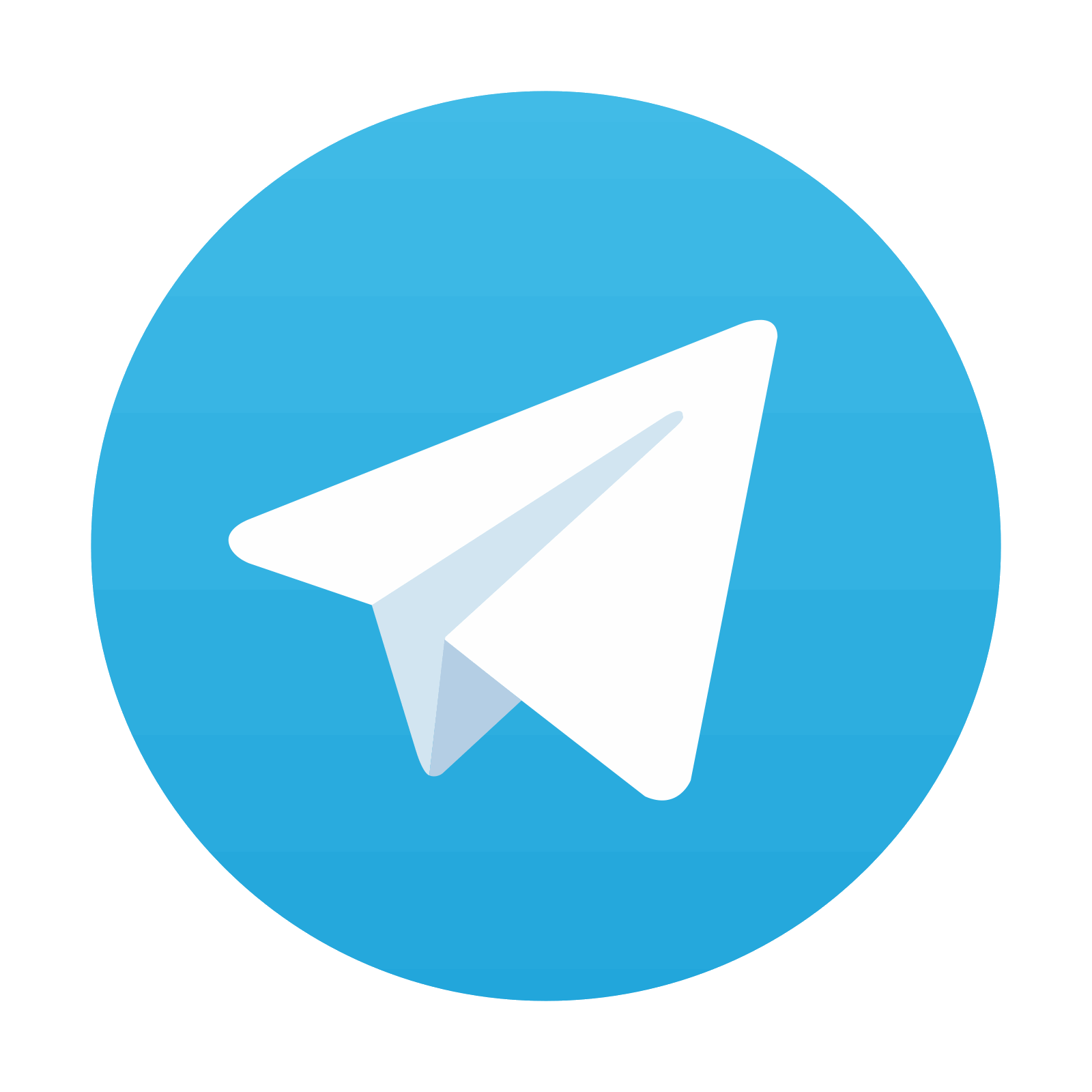
Stay updated, free articles. Join our Telegram channel
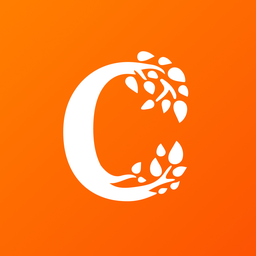
Full access? Get Clinical Tree
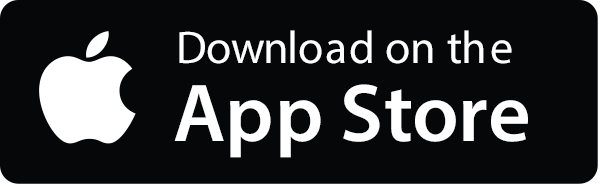
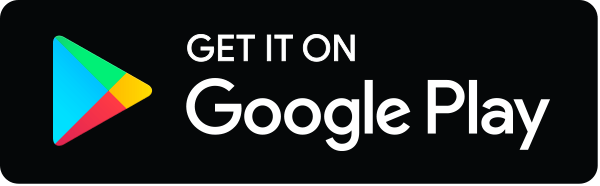
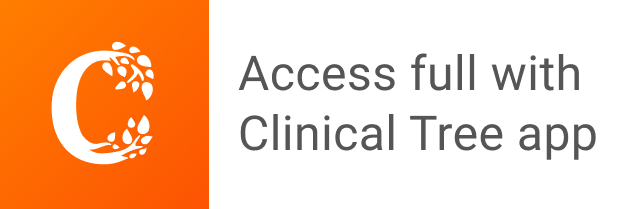