Despite significant efforts in prevention and treatment, coronary artery disease (CAD) remains the leading cause of death in the United States, accounting for one in every seven deaths. Each year nearly 700,000 Americans are estimated to have a new myocardial infarction (MI), and nearly 325,000 to have a recurrent infarction. Furthermore, an additional estimated 165,000 will have their first silent MI. The current clinical “gold standard” for the diagnosis of significant (≥50% diameter stenosis) CAD is catheter-based invasive x-ray angiography. More than a million catheter-based x-ray coronary angiograms are performed annually in the United States, with a higher volume in Europe. However, these invasive tests have a relatively low yield, with less than 40% of patients referred for x-ray coronary angiography having obstructive CAD, unnecessarily exposing these patients to the potential risks and complications of an invasive test that includes ionizing radiation and iodinated contrast. To relieve symptoms or decrease pharmaceutical use, percutaneous coronary intervention in single vessel disease is commonly performed, but the greatest impact on mortality occurs with mechanical intervention among patients with left main (LM) and multivessel CAD. Thus alternative noninvasive imaging modalities, which allow direct visualization of the proximal and mid native coronary vessels for the accurate identification/exclusion of LM/multivessel CAD, are desirable.
Cardiovascular magnetic resonance (CMR) imaging is a very promising noninvasive tool for comprehensive early risk assessment, guidance of therapy, and treatment monitoring of CAD. CMR is considered the gold standard for the assessment of cardiac anatomy, biventricular systolic function, myocardial viability (late gadolinium enhancement [LGE]) and rest/stress myocardial perfusion. Clinical research studies also have demonstrated its usefulness for quantitative myocardial tissue characterization (T 1 and T 2 relaxation time mapping), and its ability to differentiate between healthy and diseased tissue. Coronary magnetic resonance imaging (MRI) is a noninvasive diagnosis alternative to catheter-based x-ray angiography among patients with suspected anomalous coronary artery disease and coronary artery aneurysms. Although coronary multi-detector computed tomography (MDCT) offers superior isotropic spatial resolution and more rapid imaging, coronary MRI has advantages over MDCT in several respects, including the absence of ionizing radiation or iodinated contrast, which facilitates follow-up scanning, as well as smaller artifacts related to epicardial calcium. Because of the advantages of coronary MRI and its diagnostic accuracy, coronary MRI is recommended and deemed appropriate in patients suspected of anomalous coronary artery disease by both the American College of Cardiology and American Heart Association. However, CMR assessment of coronary lumen integrity and plaque burden/activity remains challenging. Nevertheless, CMR has shown great potential for coronary lumen, plaque (with and without contrast agents) and thrombus/hemorrhage visualization. The combination of these techniques could add invaluable prognostic information for patients at risk or with known CAD. Thus technical developments are being investigated to allow coronary MRI to achieve similar diagnostic lumen accuracy as the current clinical gold standard, as well as plaque characterization. Main technical challenges include suboptimal spatial resolution (as a result of long acquisition times required), and coronary motion suppression with unpredictable scan times (depending largely on the breathing pattern of the subject). In this chapter, we will review the technical imaging strategies for MRI of coronary arteries, coronary vessel walls, and coronary veins. The clinical results of coronary MRI are addressed in Chapter 24 .
Coronary Magnetic Resonance Imaging
The early approaches to coronary artery MRI were based on two-dimensional (2D) breath-hold electrocardiogram (ECG)-triggered segmented sequences. Subsequently, three-dimensional (3D) free-breathing approaches have replaced 2D breath-hold approaches, enabling greater anatomical coverage and higher signal-to-noise ratio (SNR). Three-dimensional coronary MRI can be acquired using a targeted or whole-heart coverage of the coronary anatomies. In the targeted technique, a double-oblique 3D volume aligned along the major axis of the left or right coronary artery (RCA) is acquired. For the visualization of the RCA, the imaging plane passing through the proximal, mid, and distal coordinates of the RCA is identified and the targeted 3D coronary sequence is acquired in this orientation, typically with a 30-mm slab with 20 slices, using a segmented acquisition. For the imaging of the left main (LM), left anterior descending (LAD), and left circumflex (LCX) coronary arteries, a 3D volume is interactively prescribed in the axial plane centered about the LM coronary artery ( Fig. 23.1 ). In the whole-heart coronary MRI technique, an axial (or coronal) 3D volume encompassing the entire heart is sampled in a single acquisition, in a manner analogous to coronary MDCT. This facilitates the imaging setup via simpler slab prescription, and provides a more complete anatomical coverage, positioned ~1 cm above the LM and extending to the inferior cardiac border. However, based on single-center trials to date, it has not been shown to be superior to the targeted approach for CAD assessment ( Table 23.1 ).
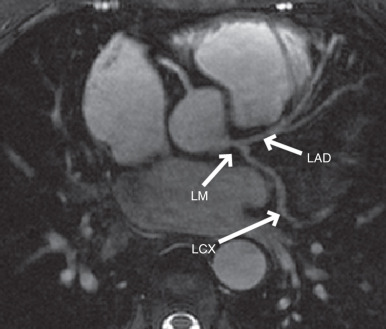
Study | Single-Center/Multicenter | No. of Patients | Sensitivity | Specificity |
---|---|---|---|---|
Noncontrast 3D Targeted Coronary MRI | ||||
Kim | Multicenter | 109 | 88%–98% | 32%–52% |
Bunce | Single-center | 46 | 50%–89% | 72%–100% |
Sommer | Single-center | 107 | 74%–88% | 63%–91% |
Bogaert | Single-center | 21 | 85%–92% | 50%–83% |
Noncontrast 3D Whole-Heart Coronary MRI | ||||
Jahnke | Single-center | 21 | 79% | 91% |
Sakuma | Single-center | 39 | 82% | 91% |
Sakuma | Single-center | 131 | 82% | 90% |
Pouleur | Single-center | 77 | 100% | 72% |
Kato | Multicenter | 138 | 88% | 72% |
Contrast Enhanced 3D Whole-Heart Coronary MRI | ||||
Yang | Single-center | 62 | 94% | 82% |
Yang | Multicenter | 272 | 91% | 80% |
Targeted thin-slab 3D acquisitions have been acquired using both gradient recalled echo (GRE) and balanced steady-state free precession (bSSFP) sequences. A thin-slab 3D targeted acquisition with a GRE sequence results in more homogenous blood pool signal, but is heavily dependent on the inflow of unsaturated protons. Saturation effects will cause a local signal loss if coronary artery flow is slow or stagnant. This signal loss is often relatively exaggerated, as compared with the lumen stenosis. Compared with GRE sequences, bSSFP provides intrinsically higher SNR because of its balanced gradients and improved blood-myocardium contrast attributed to its T 1 /T 2 weighting, with reduced sensitivity to inflow effects. Both GRE and bSSFP have been used for targeted 3D coronary MRI, where both have shown similar diagnostic accuracy for CAD. For whole-heart noncontrast coronary MRI at 1.5 T, SSFP appears to be the sequence of choice as a result of its higher blood–myocardium contrast and superior inflow properties.
Even with these technical advances, clinical acceptance of coronary MRI remains challenging because of coronary artery motion, long scan times, limited spatial resolution, suboptimal SNR and blood–myocardium contrast-to-noise-ratio (CNR). The technical challenges in coronary artery MRI are different from other CMR acquisitions as a result of unique issues including the coronary artery: (1) small caliber (3–6 mm diameter), (2) high level of tortuosity, (3) near-constant motion during both the respiratory and the cardiac cycles, and (4) surrounding signal from adjacent epicardial fat and myocardium.
Cardiac-Induced Motion
Bulk epicardial coronary artery motion is a major impediment to coronary CMR, and it can be separated into motion related to direct cardiac contraction/relaxation during the cardiac cycle and motion attributed to superimposed diaphragmatic and chest wall movement during respiration. The magnitude of motion from each component may greatly exceed the coronary artery diameter, leading to blurring artifacts in the absence of motion–suppressive methods.
To compensate for bulk cardiac motion, accurate external ECG synchronization with QRS detection is required, and vector ECG approaches are preferred. Coronary artery motion during the cardiac cycle has been characterized using both catheter based x-ray angiography and CMR. Both the proximal/mid RCA and the LAD display a triphasic pattern, with the magnitude of in-plane motion nearly twice as great for the RCA. Coronary artery motion is minimal during isovolumic relaxation, approximately 350 to 400 ms after the R wave, and again at mid diastole (immediately before atrial systole). The duration of LAD diastasis is longer than that of the RCA, and it begins earlier in the cardiac cycle. The duration of the mid diastolic diastasis period is inversely related to the heart rate and dictates the preferred coronary artery data acquisition interval.
As compared with MDCT in which the acquisition is constrained by gantry rotation, for coronary artery MRI the acquisition interval is adapted to the heart rate/diastasis interval using a patient-specific diastasis period. This can be readily identified by the acquisition of high temporal resolution cine dataset orthogonal to the long axis of the proximal/mid RCA and of the LAD. Semiautomated tools to identify the optimal data acquisition window have also been proposed. For patients with a heart rate of 60 to 70 beats per minute, a coronary artery MRI acquisition duration of ~80 ms during each cardiac cycle results in improved image quality. The duration must be further abbreviated (e.g., <50 ms) at higher heart rates, whereas with bradycardia, the acquisition interval can be expanded to 120 ms or longer. The use of patient-specific acquisition windows serves to reduce overall scan time. Image degradation can be caused by sinus arrhythmia, leading to heart rate variability, which is common especially in younger adults. An adaptive real-time arrhythmia rejection algorithm can correct for heart rate variability and improves coronary artery MRI quality.
Respiratory-Induced Motion
The second major challenge for coronary artery MRI is compensation for bulk respiratory motion. With inspiration, the diaphragm may descend up to 30 mm and the chest wall expands, resulting in an inferior displacement and anterior rotation of the heart. Several approaches have been proposed to minimize respiratory motion artifacts, including sustained end-expiratory breath-holding, chest wall bellows, respiratory navigators, fat navigators, and self-gating methods.
Prolonged (15–20 seconds) end-expiratory breath-holds were used to suppress respiratory motion in initial 2D coronary artery MRI methods. Breath-holding offers the advantage of relative ease of implementation in compliant subjects, but it limits the temporal acquisition window, image spatial resolution, and anatomic coverage. Additionally, many patients are unable to adequately sustain a breath-hold. Furthermore, slice registration errors (attributed to variability in end-expiratory diaphragmatic position) are very common as is diaphragmatic drift during the breath-hold and may occur in up to half of patients. Supplemental oxygen and hyperventilation (separately or in combination) can be used to prolong the breath-hold duration, but these methods may not be appropriate for all patients, and both diaphragmatic drift and slice registration errors persist.
Diaphragmatic respiratory navigators, first proposed by Ehman for abdominal MRI, enable free-breathing acquisitions without the stringent time constraints and patient cooperation requirements imposed by multiple breath-holds, and thus offer superior spatial resolution opportunities. Although the specifics of navigator implementation vary among CMR vendors, in the ideal implementation, the navigator can be positioned at any interface that accurately reflects respiratory motion, including the dome of the right hemidiaphragm ( Fig. 23.2 ), the left hemidiaphragm, the anterior chest wall, the anterior free wall of the left ventricle, or even through the coronary artery of interest. The navigator should not cause an image artifact and should be temporally located immediately preceding the imaging portion of the sequence with data accepted (used for image reconstruction) only when the navigator indicates that the “interface” (e.g., diaphragm position) falls within a user-defined window. The dome of the right hemidiaphragm has become the preferred location because of the simplicity and ease in set-up, where the motion of the right hemidiaphragm in the superior-inferior direction can be tracked. From CMR studies of cardiac border position during the respiratory cycle, it was observed that the ratio between cardiac and diaphragmatic displacement is ~0.6 for the RCA and ~0.7 for the left coronary artery at end-expiration, although there is variability among subjects and position (e.g., supine vs. prone imaging). This rule-of-thumb offers the opportunity for prospective navigator gating with real-time tracking, in which the position of the interface (diaphragm) is determined, and the slice position coordinates can then be shifted in real time (before the data collection) to appropriately adjust spatial coordinates. This technique allows for the use of wider gating windows and increased navigator efficiency, leading to shorter scan times. Real-time tracking implementations with a 5-mm diaphragmatic gating window are often used with a navigator efficiency approaching 50%. Coronary artery MRI with real-time navigator tracking has been shown to minimize registration errors (as compared with breath-holding) while maintaining or improving the image quality. It should also be noted that the quality of coronary artery MRI is improved by using consistent ECG timing, as well as respiratory suppression methodology for both the coronary localizing/motion scout images and for the coronary artery MRI acquisitions.
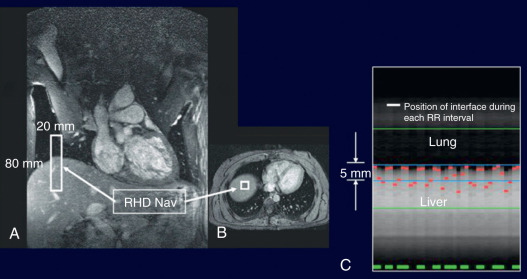
A number of refinements to the navigator method have been proposed. Although a “fixed” superior–inferior correction factor of 0.6 (with no left–right or anterior–posterior correction) is commonly used, significant individual variability has been observed. A subject-specific tracking factor has been advocated and shown to improve the quality of coronary images when the subject-specific tracking factor differs from 0.6. The use of multiple navigator locations, use of leading and trailing navigators, and use of navigators that provide guidance for affine transformations (i.e., 3D translations and rotations) of the slice prescription for each heart beat have been proposed. The affine transformation permits use of larger navigator windows, and hence higher navigator efficiency. It has also been proposed that the heart itself be tracked, such as with methods that track the epicardial fat to detect the heart position. Navigator gating with fixed scan efficiency has also been studied, which results in imaging at a fixed scan time based only on heart rate and acquisition duration. Novel k -space trajectories and various image reconstruction based methods, such as cross correlation of low resolution images, have also been proposed for respiratory motion compensation.
Self-navigation methods have also been proposed to derive the respiratory-induced motion of the heart from the acquired data itself without the need of either a one-dimensional (1D) navigator echo or a heart-diaphragm tracking factor. Respiratory-induced displacements of the heart can be directly estimated from the repetitive acquisition of the central k -space point or the central k -space line, corresponding to zero-dimensional or 1D projections of the field of view. Similar to the 1D navigator echo approaches, self-navigation methods typically perform motion correction only in the foot-head translational motion. Another drawback of the self-navigation approach is that the inclusion of static structures, such as the chest wall, can degrade motion estimation and correction.
To overcome these problems and account for more complex motion, several 2D and 3D image-based navigator (so-called iNAV) approaches have been proposed for coronary artery MRI ( Fig. 23.3 ). In these approaches a low-resolution 2D or 3D image is acquired in every heartbeat before (or after) the coronary MRI data acquisition. The main advantage of this approach is that the moving heart can be spatially isolated from surrounding static tissues and the respiratory-induced cardiac motion can be directly estimated via (rigid or affine) image-registration of iNAVs at different respiratory positions. Self-navigation and image-based navigator methods can be used to gate the acquisition and correct for motion within a small gating window, as described for 1D navigator echoes. Furthermore, because these methods directly track heart motion/position, a much larger gating window can be used, or it can be removed entirely, thereby increasing the scan efficiency to, or close to, 100%. These promising approaches may lead to shorter and predictable scan times.
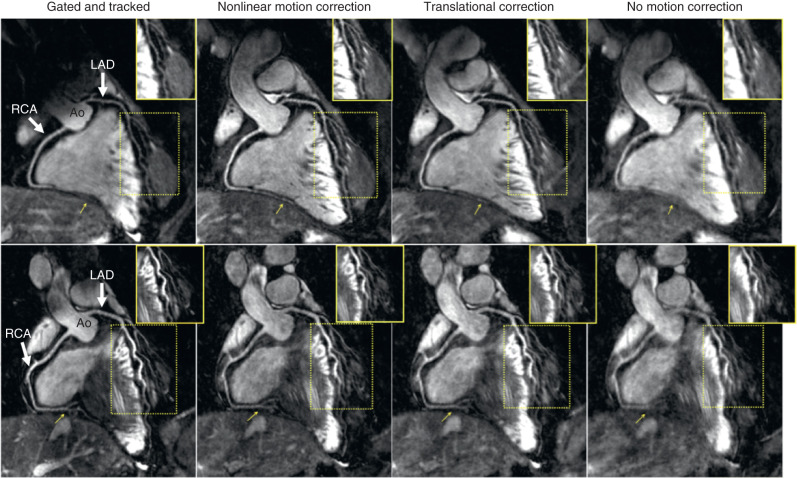
Image Quality Assessment
Epicardial fat and the myocardium surround the coronary arteries. Thus CNR can be improved by suppressing the fat and myocardium signals surrounding the coronary arteries. Frequency (spectrally) selective prepulses are applied to saturate signal from fat tissue, thereby allowing visualization of the underlying coronary arteries. To differentiate myocardium and the coronary lumen, endogenous contrast preparation techniques are commonly used. Two methods that can enhance the contrast between the coronary lumen and underlying myocardium are T2 preparation prepulses and magnetization transfer contrast (MTC). The former is often used for coronary artery MRI because it also suppresses deoxygenated venous blood, whereas the latter is used for coronary vein CMR.
The limited SNR in coronary artery MRI, along with constraints on acquisition duration, restricts the spatial resolution in the acquisition. Spatial resolution requirements for clinical coronary artery MRI depend on whether the goal is to identify the origin and proximal course of the coronary artery (e.g., issues of anomalous coronary disease) or to identify focal stenoses in the proximal and middle segments.
The SNR of coronary MRI can be enhanced by higher B 0 field strength, larger 3D spatial coverage, vasodilator administration, and contrast agents based on gadolinium chelates. The intrinsically higher SNR associated with higher magnetic field strengths may be advantageous for noncontrast coronary MRI. However, additional considerations, such as higher B 1 and B 0 inhomogeneity and higher specific absorption rate, affect certain aspects of coronary MRI, such as the diminished utility of bSSFP sequences at 3 T. Hence, GRE sequences, which are less sensitive to field inhomogeneity, as well as localized shimming and contrast preparation techniques that deal with B 1 inhomogeneities have been advocated.
The increased coverage of whole-heart coronary MRI can potentially improve the SNR, but this also increases the scan time. Thus the SNR gain is often counteracted by the need for accelerated imaging to reduce scan time, which carries an SNR penalty. Furthermore, whole-heart imaging suffers from saturation effects of the inflowing blood magnetization. Despite these issues, excellent image quality of whole-heart coronary MRI has been shown in several studies, and an example from a single-center study is depicted in Fig. 23.4 . Another technique to improve SNR in coronary artery MRI is the administration of vasodilators because the increased coronary blood flow secondary to vasodilatation reduces the inflow saturation effects. Fig. 23.5 demonstrates the impact of sublingual isosorbide dinitrate administration on 3D targeted coronary artery MRI up to 30 minutes after drug administration, in terms of subjective image quality and objective SNR and vessel sharpness.
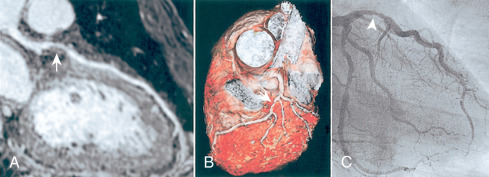
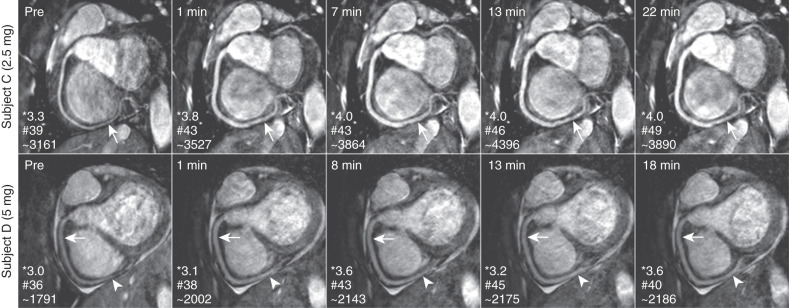
The administration of exogenous gadolinium contrast agents (both extracellular and intravascular ) that shorten the T1 relaxation time provides an alternative flow-independent approach to improve SNR and CNR. Because conventional extracellular contrast agents (e.g., gadopentetate dimeglumine [Gd-DTPA]) diffuse rapidly into the interstitial space, early contrast-enhanced coronary artery MRI studies focused on breath-hold coronary artery MRI to take advantage of the first passage of these agents. However, both the breath-hold and first-pass aspects of such approaches limit the spatial resolution and are unsuitable for whole-heart coronary acquisitions. Following the availability of a high relaxivity extracellular contrast agent, gadobenate dimeglumine (Gd-BOPTA; MultiHance; Bracco Imaging SpA, Milan, Italy), improved whole-heart coronary artery MRI at 3 T was shown to be feasible using a T1-weighted inversion recovery (IR) GRE sequence with a slow infusion of Gd-BOPTA. An example of a contrast-enhanced whole-heart coronary artery MR image from a CAD patient and the corresponding x-ray angiogram is shown in Fig. 23.6 , demonstrating agreement between two modalities in detecting significant stenosis. A bolus infusion of Gd-BOPTA for coronary MRI has also been reported, and an example depicted in Fig. 23.7 shows a clear visualization of the three major coronary vessels in the reformatted and 3D-volume rendered images. Furthermore, the bolus contrast injection method is advantageous in multiple ways because it simplifies the initiation time of coronary MRI acquisition compared with slow infusion, and it is compatible with late gadolinium enhancement imaging, which enables the assessment of coronary artery stenosis and myocardium viability using a single bolus contrast injection. Many patients with CAD also have renal dysfunction. The use of gadolinium contrast coronary MRI must consider the patient’s renal function for issues related to nephrogenic systemic fibrosis and long-term retained gadolinium.
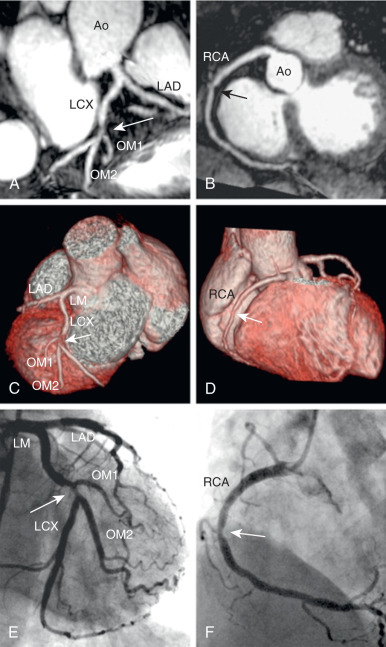
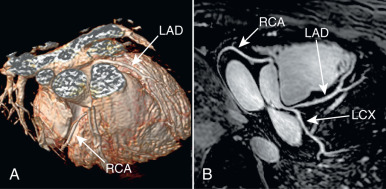
Coronary Magnetic Resonance Imaging—Advanced Methods
The sensitivity and specificity of coronary MRI for detection of CAD remain moderate, based on single-center ( Table 23.1 ) and multicenter studies (see Chapter 24 ), despite the tremendous technical improvements in the last two decades. Coronary motion, SNR and CNR remain as major impediments to coronary MRI, and these issues need to be addressed before clinical prime time for coronary MRI. To overcome some of these hurdles, several CMR centers continue with the development and implementation of novel approaches, including non-Cartesian acquisitions, accelerated imaging techniques, coronary vein MRI, and higher field imaging.
Non-Cartesian acquisitions provide efficient k -space traversals that lead to incoherent or less visually significant artifacts. Thus alternative non-Cartesian k -space acquisitions, including spiral and radial coronary MRI, have received attention. The use of spiral coronary artery MRI was first reported more than 2 decades ago. Spiral acquisitions are advantageous to Cartesian acquisitions in several respects, including a more efficient filling of k -space, enhanced SNR, and favorable flow properties. However, drawbacks of spiral trajectories include increased sensitivity to magnetic field inhomogeneity and longer image reconstruction. Interleaved spiral imaging is typically used because of reduced artifacts, although a single-shot k -space trajectory can also be employed. Both breath-hold and free-breathing/navigator-gated 2D acquisitions can be performed with spiral coronary artery. Compared with conventional Cartesian approaches, single spiral acquisitions (per RR interval) afford a near 3-fold improvement in SNR. Hence, acquiring two spirals during each RR interval will halve the acquisition time, while maintaining superior SNR (vs. Cartesian acquisition) and CNR. Variable density spirals have also shown benefit. Radial trajectories also enable more rapid acquisitions, while decreasing sensitivity to motion. Data in healthy subjects appear promising and may be particularly beneficial for coronary wall imaging.
Parallel imaging techniques such as generalized autocalibrating partially parallel acquisition (GRAPPA) or sensitivity encoding (SENSE) are the most commonly used clinical acceleration technique for coronary artery MRI. Resultant acceleration rates of up to 2-fold while using 5 to 16 element cardiac-coil arrays, and up to 4-fold acceleration rate using 32-channel coils have been achieved. Currently, parallel imaging is considered the state-of-the-art accelerated imaging technique for whole-heart coronary artery MRI, and is commonly used for clinical imaging.
In addition to the non-Cartesian trajectories described previously, compressed sensing (CS) has emerged as a robust alternative acceleration technique that exploits the sparsity of the image in a transform domain. CS also requires an incoherent undersampling pattern, which can be achieved by random undersampling of k -space data in the k y – k z plane for 3D Cartesian acquisitions. In high-resolution coronary MRI, an advanced CS-based reconstruction strategy was shown to provide reconstructions with reduced blurring compared with conventional CS techniques and was successfully used in contrast-enhanced whole-heart coronary MRI. More recently, for highly-accelerated sub-millimeter resolution whole-heart coronary MRI, CS was shown to outperform parallel imaging at 6-fold accelerated imaging in a head-to-head comparison ( Fig. 23.8 ). CS can also be used in conjunction with non-Cartesian imaging, such as with spiral acquisitions, to enable whole-heart acquisitions in a single prolonged breath-hold or with 3D radial trajectories.
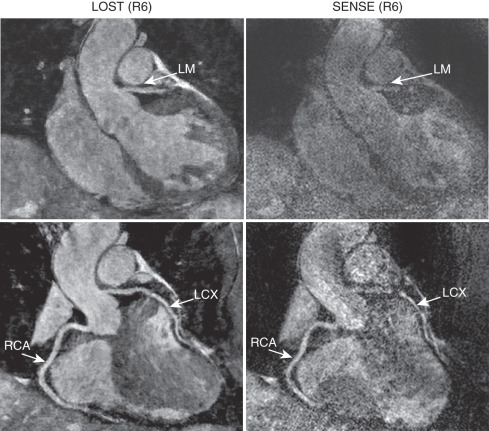
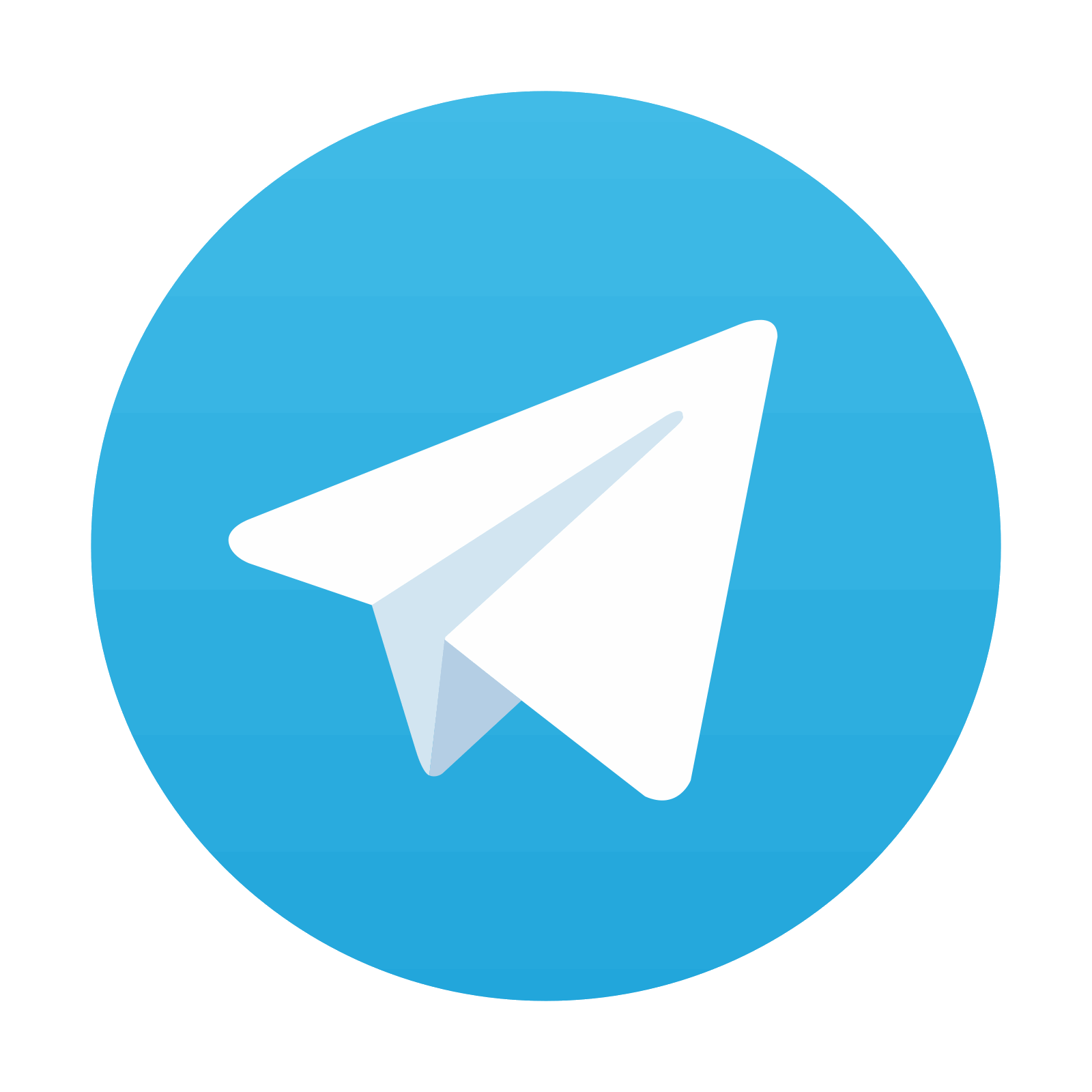
Stay updated, free articles. Join our Telegram channel
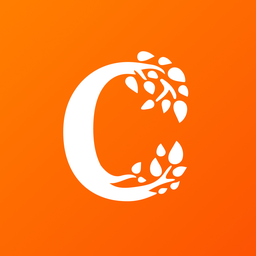
Full access? Get Clinical Tree
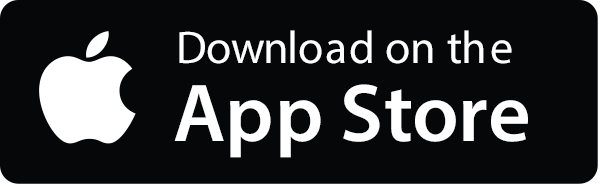
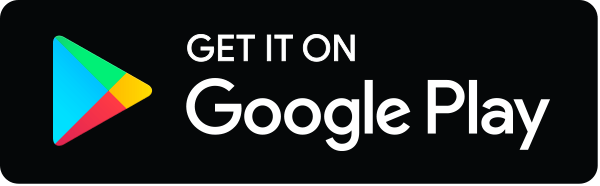
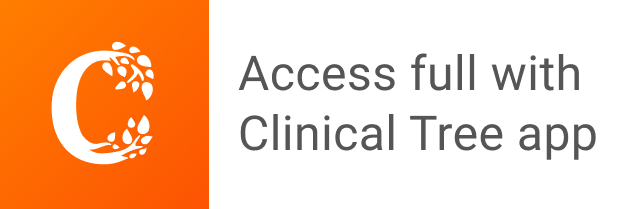