Management of Respiratory Motion in Radiation Therapy
Gig S. Mageras
Paul J. Keall
Increased interest in managing respiratory motion in radiation treatments has been prompted by several factors. First, there has been limited ability to control tumors in the thorax and abdomen with standard radiotherapy techniques. Second, anatomic movement with respiration, at least in some circumstances, limits the accuracy with which radiation can be delivered to tumor-bearing tissue. The resultant larger treatment volumes required to accommodate target mobility may limit the tumoricidal dose due to the larger amounts of surrounding normal tissue exposure, particularly for larger treatment volumes. Conversely, underestimation of the required treatment margins may result in marginal misses. Therefore, it seems desirable to limit respiratory motion for tumor sites exhibiting large excursions. Third, technologic advances have spawned new capabilities for measuring and reducing respiratory motion. This chapter discusses the problems associated with respiratory motion and surveys different strategies for managing respiratory motion during radiation treatment. A more detailed discussion can be found in the report of Task Group 76 of the American Association of Physicists in Medicine (AAPM).1
Respiratory motion affects image acquisition, treatment planning, and radiation delivery. Computed tomography (CT) images appear distorted because CT study times are long with respect to respiratory motion timescales, leading to a wrong appreciation of target and organ-at-risk (OAR) shapes and extents. The left panel of Figure 5.1 illustrates the effect of respiratory motion on standard CT acquisition.2 The object moves inferiorly-superiorly through the CT scan plane; hence, consecutive CT images occur at disordered positions in the object along the superior-inferior direction, and the object appears distorted. The right panel of Figure 5.1 illustrates this effect in a clinical image. The motion artifacts give rise to a systematic error in tumor position and extent (i.e., the tumor observed in the image is not the respiration-averaged position).3, 4, 5
Respiratory motion also limits target localization accuracy while imaging at treatment. A limitation of cone beam CT (CBCT) systems is image degradation caused by patient motion artifacts, which is a consequence of the limited gantry speed and the resultant long (approximately 1 minute) acquisition times. Respiratory motion, in particular, degrades images in the thorax and abdomen. Distortion in CBCT is completely different than for standard CT: Projection images occur at different respiration phases; thus, sagittal, coronal, and axial reconstructions are blurred, and axial images show streaking artifacts. The resultant difference between CT and CBCT leads to a systematic error in observed tumor position and shape.6
Treatment planning to account for respiratory motion poses additional problems. There is no motion information available from standard CT, so one often resorts to using generic margins rather than patient-specific ones. However, patients exhibit widely differing amounts of tumor mobility with respiration; thus, the choice of generic margins is likely to be too large or too small.
Respiratory motion during radiation delivery blurs the planned dose distribution, which in standard clinical practice is calculated without explicit inclusion of motion. For treatment fields with uniform radiation intensity within the field, respiratory motion will broaden the dose distribution in the anatomy moving near the beam edges. For fields delivered with physical compensators, the dose gradients in moving tissue will broaden and become less steep.
Intensity-modulated radiation treatment (IMRT) delivered with a multileaf collimator (MLC) poses additional considerations because of the interplay between moving MLC and respiratory motion.7 An intensity-modulated field is composed of many small fields that are delivered temporally; thus, the dose actually received by moving tissue may be less than, or greater than, the planned dose. Initial studies reported large dose variations, exceeding 20%, for a single field.8,9 Subsequent studies have found that for multiple field treatments with 30 fractions and assuming that the start of each treatment field occurs at a random point in the breathing cycle, dose variations average out to produce distributions that are the same as for treatments delivered with a physical compensator.10, 11, 12 Consideration of interplay effects should be given for limited-fraction IMRT and with scanning particle beams. Respiratory motion in liver can have a clinically important effect on proton fields (Fig. 5.2), causing underdose in the distal portion of the target volume or overdose to normal tissue beyond the distal portion.13
Interfraction variations in tumor position result in a displacement of the dose distribution. There are few published measurements on interfraction variation of tumor position in
lung because the intrafraction respiration-induced motion is more dominant and obscures the interfraction component.14,15 Interfraction variations can be decomposed into systematic and random components, similar to analyses of other geometric errors. A systematic error is one in which a quantity observed in the planning image set, such as target position, differs from its mean position over the course of treatment, whereas a random error is the deviation of the quantity from its mean position on any given treatment fraction. For a similar magnitude of error, a systematic displacement of the dose distribution has a larger effect on the dose to the target, whereas the effects of breathing motion and random displacements are smaller.6,15,16
lung because the intrafraction respiration-induced motion is more dominant and obscures the interfraction component.14,15 Interfraction variations can be decomposed into systematic and random components, similar to analyses of other geometric errors. A systematic error is one in which a quantity observed in the planning image set, such as target position, differs from its mean position over the course of treatment, whereas a random error is the deviation of the quantity from its mean position on any given treatment fraction. For a similar magnitude of error, a systematic displacement of the dose distribution has a larger effect on the dose to the target, whereas the effects of breathing motion and random displacements are smaller.6,15,16
MEASUREMENT OF RESPIRATORY MOTION
Published observations of the extent of respiration-induced organ motion include lung, esophagus, liver, pancreas, breast,
kidney, spleen, and prostate.1,17,18 Studies have tracked the movement of tumor, host organ, radiographic fiducials implanted at the tumor site, and surrogate organs such as the diaphragm, which are assumed to correlate with the tumor. Patient breathing patterns can vary in magnitude, period, and regularity during and between treatment sessions, although the breathing pattern within a session is usually more stable than between sessions (Fig. 5.3). A study of three-dimensional (3D) trajectories of fiducials implanted in or near pulmonary tumors of 20 patients revealed hysteresis in half of the cases.19 The hysteresis amounted to a 1- to 5-mm difference in the trajectories between inhalation and exhalation (Fig. 5.4).
kidney, spleen, and prostate.1,17,18 Studies have tracked the movement of tumor, host organ, radiographic fiducials implanted at the tumor site, and surrogate organs such as the diaphragm, which are assumed to correlate with the tumor. Patient breathing patterns can vary in magnitude, period, and regularity during and between treatment sessions, although the breathing pattern within a session is usually more stable than between sessions (Fig. 5.3). A study of three-dimensional (3D) trajectories of fiducials implanted in or near pulmonary tumors of 20 patients revealed hysteresis in half of the cases.19 The hysteresis amounted to a 1- to 5-mm difference in the trajectories between inhalation and exhalation (Fig. 5.4).
Recent studies have used respiration-correlated CT (RCCT; described further in the next section), which provides 3D information on anatomic position at several points in the respiratory cycle, to measure organ motion in the thorax and abdomen.20, 21, 22, 23, 24, 25 In the largest such statistical analysis, Liu et al.25 examined tumor motion in 152 patients with stage III or IV non-small-cell lung carcinoma (NSCLC). The analysis used rigid soft tissue-based image registration to measure tumor motion, which was found to be primarily in the superior-inferior (SI) direction. Ninety-five percent of tumors moved less than 1.3 cm SI, 0.4 cm left-right (LR), and 0.6 cm anterior-posterior (AP). Tumor motion was highly correlated with diaphragm excursion and tumor location in the SI direction. Brandner et al.21 reported on the motion of abdominal organs in 13 patients using RCCT. Motion was largest in the SI direction, with mean SI displacement during tidal breathing of 1.3 cm for the liver, 1.3 cm for the spleen, and 1.2 cm for the kidneys. Motion of up to 2.5 cm inferiorly was observed in all organs, and motion of up to 1.2 cm anteriorly was observed in liver and kidneys. The numerous studies indicate that patients exhibit a broad range of respiratory patterns and amplitudes of motion.
ACCOUNTING FOR RESPIRATORY MOTION AT SIMULATION
Different CT methods have been proposed for measuring respiration-induced tumor motion as a means of designing the treatment plan to encompass the motion. It is important to keep in mind that in all of these methods, respiratory patterns and, hence, tumor motion extent can change between simulation and treatment sessions.
SLOW COMPUTED TOMOGRAPHY SCANNING AND BREATH-HOLD COMPUTED TOMOGRAPHY
Slow CT scanning has been proposed for peripheral lung tumors,26 such that one or more respiratory cycles are recorded per CT slice; hence, the image of the tumor, at least when surrounded by lower density lung, shows the full extent of respiratory motion. An additional advantage is that the patient geometry is a respiration-averaged one; thus, the dose calculation is more representative of treatment than is fast CT. A disadvantage is motion blurring, which renders organ delineation in the images more difficult. In addition, the method can underestimate the motion extent of lung tumors attached to the mediastinum, chest wall, or diaphragm.
Two sets of breath-hold CT scans, one each at tidal end expiration and end inspiration, are often used to estimate tumor respiratory excursion.27 The method relies on the patient’s ability to hold his or her breath reproducibly and at the correct inspiration level; thus, some means of verifying inspiration level is desirable, such as spirometry or optical monitoring of abdominal displacement. Because the breath-hold scans will overestimate or underestimate lung volumes at treatment, a free-breathing or slow CT scan may also be acquired for treatment planning purposes.
RESPIRATION-CORRELATED COMPUTED TOMOGRAPHY/FOUR-DIMENSIONAL COMPUTED TOMOGRAPHY
RCCT, which is often referred to as four-dimensional (4D) CT, addresses both problems associated with free-breathing CT—image distortion caused by respiratory motion and lack of motion information.28, 29, 30, 31 The approach common to these methods is to acquire sufficient data for generating CT images at all phases of the respiratory cycle while simultaneously recording respiration, then retrospectively correlating the CT images with phase.
The concept of using cine CT acquisition for correlating with respiration was reported independently by Low et al.,29 who used spirometry for generating a respiratory signal, and Pan et al.,30 who used abdominal displacement. In both approaches, repeat CT images are acquired over slightly more than one respiratory cycle with the couch stationary while recording patient respiration; the couch is then incremented, and the process is repeated (Fig. 5.5). Following acquisition, the images are sorted with respect to the respiratory signal, leading to a set of volume images at different respiration points in the cycle.
Helical acquisition is performed by substantially reducing the pitch routinely used to approximately 0.1 to 0.5 and adjusting the acquisition parameters such that the CT beam will be on for at least one respiratory cycle at each couch position. This means that the couch velocity is much lower than for a conventional scan. With the CT beam on for at least one respiratory cycle at each couch position, there is sufficient oversampling of the respiratory cycle to create images, either reconstructed during acquisition or retrospectively using sinogram interpolation, at a number (typically eight to ten) of different states of the respiratory cycle. RCCT was originally demonstrated on single-slice scanners in helical mode.22,28,31 Subsequently, helical mode multislice RCCT acquisition has been demonstrated32,33 and is now commercially available.
Figure 5.6 shows an early clinical example of RCCT using cine acquisition. Artifacts are clearly reduced compared to standard CT, and anatomic motion is evident; however, some artifacts are still present, as indicated by the arrows, particularly at mid-expiration and mid-inspiration where motion is most rapid. Artifacts can be partly caused by too few samples per cycle. At a given desired point of reconstruction, at each table position, one chooses the image occurring at the nearest neighboring phase. Thus at each table position, the nearest neighbor image occurs at a slightly different phase. Early RCCT protocols with ten images per table position often caused artifacts. Current cine CT protocols acquire 20 to 25 images per table position to minimize this effect but at a price of typically 10 times the imaging dose of a standard CT, even with a reduced tube current.
In some commercial systems, CT images are sorted based on a respiration phase angle assigned by an algorithm that determines the period behavior of the respiratory signal. Phase-based sorting assumes repeatable breathing cycles (i.e., that the anatomy is at the same position for a given phase in every cycle). It has been recognized early on that this assumption is known to be violated for normal breathing, with its varying cycle-to-cycle amplitude variations, and has led to investigations into sorting based on the displacement of the
respiratory signal, in what is termed displacement-based sorting.34, 35, 36, 37, 38 Figure 5.7 shows the respiratory signal (tidal volume) of a patient that exhibits considerable variation in amplitude among breath cycles. Data points selected at end inspiration based on displacement (blue points in upper row) occur at similar values of tidal volume, whereas tidal volumes of data points based on phase (red points in lower row) show large variations. The resulting images reconstructed with displacement sorting (Fig. 5.8, upper row) show less artifacts than those with phase sorting (lower row).
respiratory signal, in what is termed displacement-based sorting.34, 35, 36, 37, 38 Figure 5.7 shows the respiratory signal (tidal volume) of a patient that exhibits considerable variation in amplitude among breath cycles. Data points selected at end inspiration based on displacement (blue points in upper row) occur at similar values of tidal volume, whereas tidal volumes of data points based on phase (red points in lower row) show large variations. The resulting images reconstructed with displacement sorting (Fig. 5.8, upper row) show less artifacts than those with phase sorting (lower row).
However, displacement-based correlation also has its limitations with irregular breathing. There may be no data available for a given displacement at some table positions, for example, during the shallow peaks in the upper row of Figure 5.7. A recent area of investigation has been the development of methods to further reduce irregular breathing artifacts in RCCT image sets by using deformable image registration to interpolate or extrapolate from (relatively) artifact-free images in the RCCT set.39
![]() Figure 5.8. Images reconstructed using displacement-based sorting (upper row) and phase-based sorting (lower row) for the patient corresponding to Figure 5.7. Columns from left to right: end expiration, mid-inspiration, end inspiration, and mid-expiration. Arrows indicate locations of artifacts in the phase-sorted images. (From Lu W, Parikh PJ, Hubenschmidt JP, et al. A comparison between amplitude sorting and phase-angle sorting using external respiratory measurement for 4D CT. Med Phys. 2006;33:2964-2974, with permission.) |
RESPIRATORY MOTION MEASUREMENT WITH POSITRON EMISSION TOMOGRAPHY AND MAGNETIC RESONANCE IMAGING
A number of different approaches have been examined to reduce the effects of respiratory motion on image quality in positron emission tomography (PET). Respiratory motion causes image blurring, reducing the signal-to-background contrast, decreasing the activity concentration per voxel within the lesion, and causing overestimation of lesion size. An initial approach to reducing motion is to use respiration-triggered or gated PET, in which PET data are acquired into discrete bins within each respiratory cycle.40 Clinical examples of gated PET have demonstrated its ability to reduce apparent lesion size and increase activity concentration (Fig. 5.9), albeit at the cost of reduced counts in each bin. Respiration-correlated PET has also been demonstrated, yielding similar results to gated PET but with the advantage of increased flexibility through retrospective data sorting.41 A disadvantage is that it requires considerably more computer memory and processing time than does gated PET. More recent approaches have studied deblurring of PET images by deconvolution methods,42 using deformable registration to fuse respiration-binned PET data into a single image43,44 and incorporating patient-specific motion model into PET image reconstruction.45
The effects of respiratory motion can degrade the accuracy of free-breathing CT-derived attenuation corrections on combined PET/CT units, resulting in biased activity estimates in the PET images.46 The use of RCCT for applying attenuation corrections to gated PET images, in which CT-derived attenuation corrections correspond to the respiratory bin of the PET image, has been shown to improve co-registration between PET and CT images.47,48 In situations where respiration-averaged PET is standard clinical practice, use of respiration-averaged CT for calculating attenuation corrections improves tumor identification in PET images.49 For diagnostic imaging practice, Nehmeh et al.50 have reported on a deep inspiration breath-hold (DIBH) technique for acquiring PET/CT acquisition at a single breathing phase (Fig. 5.10).
Fast magnetic resonance imaging (MRI) acquisition techniques have been used for dynamic visualization of respiratory motion in the thorax and abdomen (Fig. 5.11).51, 52, 53 An advantage over RCCT is the continuous imaging over multiple breath cycles without ionizing radiation, thereby providing an assessment of variability in tumor mobility. von Siebenthal et al.54 describe a method of respiration-correlated volumetric MRI, using anatomic structures detected in a two-dimensional (2D) image (e.g., a sagittal slice at one location) for retrospectively sorting dynamic 2D images over the patient volume (Fig. 5.12).
FOUR-DIMENSIONAL PATIENT MODELING AND TREATMENT PLANNING
RCCT has several applications, including tumor motion measurement and motion management decision making, planning for respiratory-gated radiotherapy, planning for motion-inclusive radiotherapy, and planning for tumor tracking radiotherapy. In all such applications, it is important to note that even though useful motion information is included in the RCCT, the scan typically only includes information from a single breathing cycle per anatomic position. The breathing pattern will change with time, the tumor will change position with respect to the skeletal anatomy and other organs during a course of treatment, and tumor may change size and shape, all of which need to be accounted for with an appropriate treatment strategy.
The application of RCCT for tumor motion measurement is described earlier. However the magnitude of the observed motion can affect clinical decision making. If there is little motion observed or little normal tissue sparing advantage (e.g., constant cardiac-target distance with respiratory phase), explicit respiratory motion management procedures, such as gated or 4D planning techniques, may not be necessary. The AAPM Task Group 76 report1 gives a guideline that, based on other errors in radiotherapy, for motion less than 5 mm, explicit respiratory motion management may not be necessary. Figure 5.6 in the AAPM report gives a clinical decision process for a patient for whom respiratory motion is a concern during the radiotherapy process.
Planning for motion-inclusive, respiratory-gated, and tumor tracking radiotherapy can be put in a common theoretical framework, shown schematically in Figure 5.13. The mathematical description of the problem is to find the motion sequence M as a function of phase θ, M(θ), and gating beam on/off status H(θ), where the dose distribution to be delivered, D, is

where λ(θ) is the time spent in each respiratory phase, d is the dose distribution computed on each phase, I(θ) is a CT set for a given RCCT phase, Iref is the image set used as a reference, and u(θ) is the deformable image registration vector field linking anatomy in the different image sets. The vector field is particularly useful for 4D planning because it allows information related to the anatomy to be transferred between image sets of the 4D CT scan. For example, contours drawn on one phase can be transferred to the appropriate anatomy on other phases, or dose distributions from phases can be deformably summed to estimate the composite dose. The use of vector fields to automatically compute contours on subsequent phases or sum dose distributions is necessary given that 4D CT has an order of magnitude more data and an order of magnitude more manual effort in the absence of vector fields. However, deformable registration algorithms are an area of intense study, and variations with human observers invariably exist. The algorithms should be used with caution. At the time of press, deformable registration algorithms are not widely available. However, it is likely that in 2 to 3 years, all major treatment planning system vendors will offer some form of deformable registration to facilitate 4D planning.
MOTION-INCLUSIVE TREATMENT PLANNING
Most treatment rooms do not currently have respiratory motion management devices. Therefore, treatment planning solutions are required for these situations. Based on the RCCT scan, a common approach is to determine the gross tumor volume (GTV) in each phase or a subset of the scan phases. The final GTV used for further treatment planning is the combination of each of the individual phases.55,56 Some researchers have noted that it may not be necessary to cover the full extent of tumor motion to derive an appropriate plan using inverse techniques.57 As with all respiratory motion management methods, the variation of breathing from cycle to cycle and the potential change in tumor position from day to day with respect to bony anatomy are challenges that warrant additional image guidance procedures.
RESPIRATORY-GATED TREATMENT PLANNING
For respiratory-gated delivery,58,59 often the respiratory phases to be used for treatment are chosen based on a review of the RCCT, and phases in which the target position are in similar positions are used to compute both the treatment plan and duty cycle (delivery efficiency with gating). The duty cycle is typically planned to be 30% to 50%. During delivery, depending on breathing variability, the actual duty cycle, which is determined by the ratio of gate duration to the respiratory period, can vary from that planned. Often for thoracic and abdominal tumors, the exhalation phases are used for the treatment plan and treatment because the typical patient will spend more time in exhalation than inhalation and the exhalation position is more reproducible than inhalation. The advantage to gating at inhalation is that critical structures are further from the treatment field. During inhalation, the heart moves inferiorly and posteriorly, and thus, gated breast treatments at inhalation can reduce cardiac dose. Also, the lung expansion at inhalation (and especially for deep inhalation) means that the fraction of lung irradiated for a given beam aperture is smaller at inhalation than for other phases (discussed further later in this chapter).
MOTION-ENCOMPASSING TREATMENT METHODS
Depending on clinical treatment goals, it may be sufficient not to take any measures to control respiratory motion during treatment, but instead to use motion-encompassing treatment methods. In these circumstances, it is important that respiratory motion is properly accounted for during imaging for treatment planning, as discussed in the previous sections, as well as during imaging at treatment. Chapter 3 discusses margin determination.
A common approach in commercial systems is to define a target volume that encompasses the range of tumor excursion in all images of the RCCT. Software tools allow the user to loop through reconstructed slices in the three principal directions to evaluate the encompassing target volume. For lung tumors, a maximum intensity projection (MIP) tool is available in some systems, which produces an image in which each voxel is set to the maximum CT number for that voxel in the RCCT image set (Fig. 5.14).60 The MIP image provides a tumor motion-encompassing volume in situations where the tumor is surrounded by low-density lung tissue but will underestimate the encompassing volume in locations where the tumor is attached to mediastinum, chest wall, or diaphragm (Fig. 5.15).
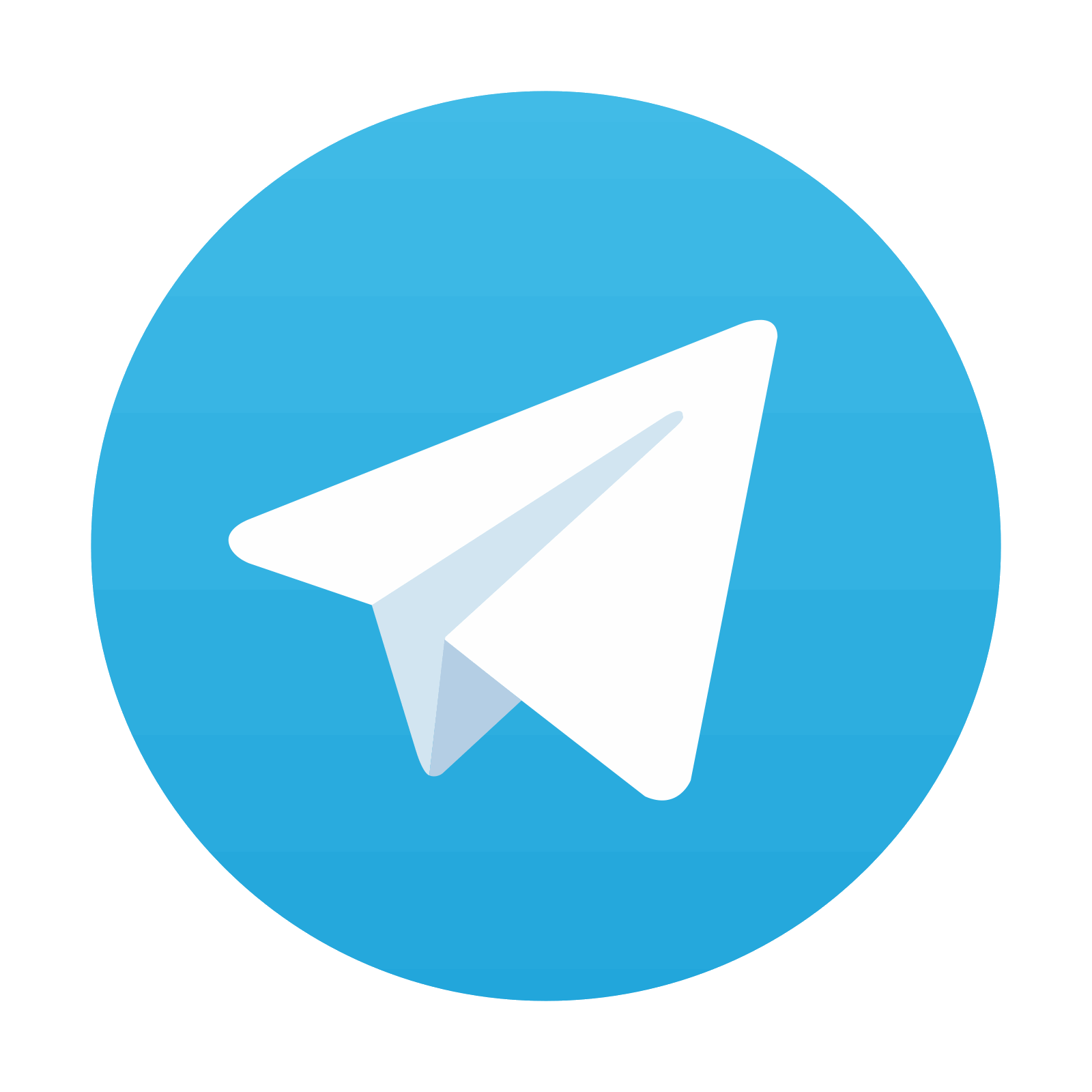
Stay updated, free articles. Join our Telegram channel
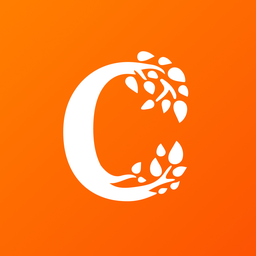
Full access? Get Clinical Tree
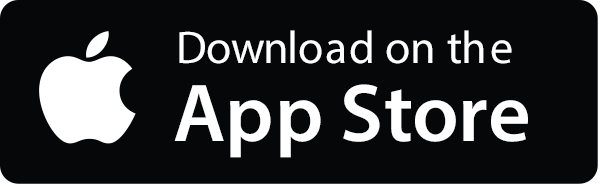
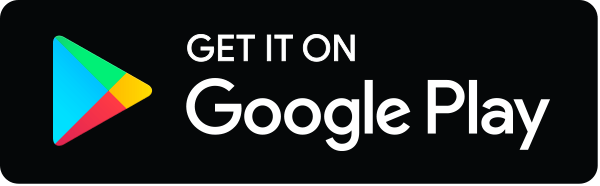