Fig. 16.1
The Blood-Brain Barrier (BBB) is composed of endothelial cells that are connected to each other via tight junctions. The endothelial cells are supported by the cells of the neurovascular unit, including pericytes, astrocytes, neurons and the basement membrane
In combination, these neurovascular unit components work to form a functional BBB that can maintain the delicate microenvironment required for the neuronal population. The BBB regulates ion and neurotransmitter ranges within a very narrow range, and also prevents the entry of pathogens from the peripheral system. The barrier is essential for preventing damage to the brain since the brain itself has very limited ability to repair after injury.
While the BBB is crucial for maintaining health, it is also a serious obstruction to pharmacological agents that could repair the brain after injury or disease. Lipid-soluble drugs smaller than 400 Da are able to cross the barrier; however, this means that over 98 % of potential small-molecule and 100 % of large molecule therapeutics potentially effective for treating brain disorders, are unable to pass the BBB in appreciable quantities (Pardridge 2005). The BBB is also equipped with ATP-binding cassette (ABC) efflux transporters, such as p-glycoprotein and multidrug resistance protein. These active transporters ensure that any toxic or foreign molecules, including therapeutic agents, are shuttled back across the BBB. The prevalence of brain diseases, including neurodegenerative diseases, brain tumors and psychiatric illnesses, is increasing at alarming rates, and currently there are very few effective treatments. This is primarily due to the presence of the BBB.
16.3 Bypassing the BBB for Effective Drug Delivery
There are limitations to the methods used to overcome the BBB for drug delivery. In some clinical trials, investigators have attempted to directly inject the drug into the targeted brain region using MRI and neuronavigation systems to position needles and pumps (Gross et al. 2011; White et al. 2010). Although this method of drug delivery is effective, the potential risks and undesirable surgical procedures prevent patients from receiving these treatments. Other methods to bypass the BBB include pharmacological modification of the drug targets in order to increase uptake through the barrier. One successful example of this technique is the addition of cysteine, a small neutral amino acid, to a non-transportable drug. This makes the drug appear to be a large amino acid, thereby allowing it to be taken up into the BBB (Killian et al. 2007). Although drug modification can be effective for bypassing the BBB, there are challenges in achieving therapeutic concentrations in the target region.
As an effort to address the limitations regarding drug delivery methods, researchers have attempted to find new ‘non-invasive’ methods to circumvent the BBB. Recent successes with intranasal delivery of therapeutic agents have gained attention. The highly vascularized nasal mucosa is adjacent to the cerebrospinal fluid (CSF), thus rapid blood concentrations can lead to therapeutic CSF drug concentrations. It has been suggested that drugs travel to the CSF via the olfactory neuroepithelium or via neuronal transport (Pires et al. 2009). Recent studies have shown that fluorescent tracers delivered intranasally may move through the perivascular spaces to reach the brain (Lochhead and Thorne 2012). These experiments have been promising in rodent models, but may be difficult to validate in humans where drug penetration throughout large brain regions is limited (Pardridge 2012).
Focused ultrasound (FUS) has been used to non-invasively and transiently open the BBB to allow drugs to pass from the blood stream into the brain (Hynynen et al. 2001). FUS has been effective as a method for delivering small and large therapeutic agents in preclinical disease models. The first clinical trials are rapidly approaching, and FUS has the potential to revolutionize drug delivery methods and be the first step to effective treatment of brain disorders.
16.4 Focused Ultrasound (FUS)-Mediated Barrier Opening
The use of focused ultrasound in the brain has been investigated since the 1950s (Fry and Fry 1960). It was first observed that heating of brain tissue through application of continuous ultrasound could result in BBB opening, but this was often accompanied by hemorrhage (Bakay et al. 1956; Shealy and Crafts 1965) or tissue coagulation (Patrick et al. 1990). At the time, both thermal- and cavitation-related mechanisms were thought to contribute to BBB opening. Further studies have confirmed that thermal mechanisms can lead to increased drug uptake by endothelial cells (Cho et al. 2002), but that thermally-induced BBB opening has so far been accompanied by tissue damage (McDannold et al. 2004). As an alternative, cavitation-related BBB opening has been explored using pulsed ultrasound application. Cavitation refers to ultrasound-induced generation, oscillation, and in its extreme form, subsequent collapse of bubbles within a tissue. Similar to thermal mechanisms, in the 1980s and 1990s, cavitation-mediated ultrasound-induced BBB opening was unpredictable and often related to damage of the surrounding blood vessels and brain tissues (Vykhodtseva et al. 1995). Several parameters were investigated, and in general it was observed that tissue damage occurred more often as pulse duration, number of pulses and repetition frequency increased (Vykhodtseva et al. 1995; Mesiwala et al. 2002).
In 2001 it was demonstrated for the first time that preformed microbubbles, delivered intravenously, could be used as cavitation nuclei, thereby reducing the ultrasound energy required to induce BBB opening, producing much more consistent results (Hynynen et al. 2001). When the circulating microbubbles pass through the focal area of the ultrasound, they concentrate the acoustic energy inside the blood vessel. The ultrasound energy causes the microbubbles to expand and contract at the frequency of the ultrasound, a process known as stable cavitation (Fig. 16.2).
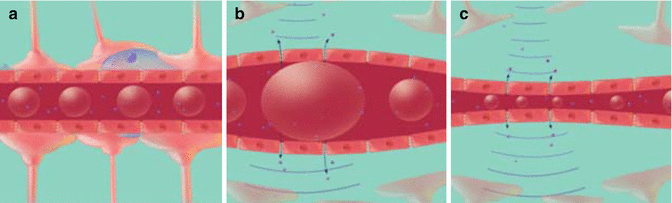
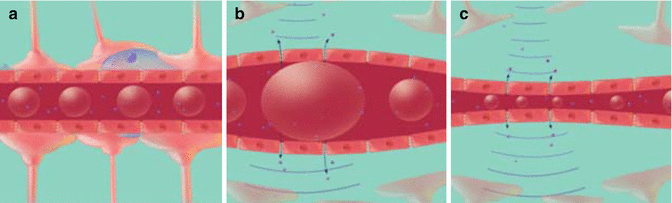
Fig. 16.2
(a) Microbubbles flow through the vasculature. (b) When ultrasound is applied, the microbubbles expand and (c) contract at the frequency of the ultrasound. The expansion and contraction of microbubbles leads to BBB opening
It is important to note the considerable differences between the high pressure generation and collapse of bubbles observed during the initial attempts at ultrasound-mediated BBB opening (Vykhodtseva et al. 1995), and then the stable oscillation of preformed bubbles that was introduced in 2001 (Hynynen et al. 2001). The addition of microbubbles has reduced the amount of ultrasound energy required to open the BBB by 100-fold. Since less energy is required, the risk of skull heating by the ultrasound is reduced, thereby making clinical treatments feasible. Also, at lower ultrasound pressures, the risk of brain tissue hemorrhage and damage is significantly reduced. Instead, the gently oscillating microbubbles interact with the endothelial cells and stimulate the opening of the tight junctions and increase transcellular molecular transport, leading to safe and effective drug delivery to the brain (Hynynen et al. 2001, 2006).
Following this first study, several groups have reported effective drug delivery using different ultrasound parameters in the presence of microbubbles. The factor that most significantly affects the amount of BBB opening is the ultrasound pressure. There is a delicate balance between applying enough ultrasound pressure to induce BBB opening for drug delivery, but limiting the ultrasound to avoid damage to the vessels and surrounding brain tissue. As pressure increases, the amount of microbubble expansion and contraction also increases, but too much pressure can lead to microbubble collapse and ensuing tissue damage. The relative amount of BBB opening required is based on the relative size of the therapeutic agent that needs to cross the BBB. Recent studies have demonstrated that greater ultrasound pressure is required to allow a 2000 kDa drug to cross the BBB, compared to the ultrasound pressure required for a 70 kDa drug (Chen and Konofagou 2014). The threshold of ultrasound pressure required for BBB opening is related to the mechanical index, defined as the peak negative pressure in-vivo by the square root of the frequency (McDannold et al. 2008a, b).
In rodents, ultrasound frequencies ranging from 28 kHz (Liu et al. 2010a, b) to 8 MHz (Bing et al. 2009) have been used to open the BBB. The size of the focal spot (or the treatment area) decreases with higher frequency, so conceptually, a higher frequency transducer would be better suited for targeting small brain nuclei. However, higher ultrasound pressures are required to induce opening when higher frequencies are used (McDannold et al. 2008a, b), therefore, the clinically relevant frequency range for therapeutic ultrasound is likely between 0.2 and 1.5 MHz. In addition to frequency, the duration of the ultrasound burst and the frequency of the burst repetitions have both been investigated to better understand their effects on BBB opening. Ultrasound burst length ranging from a few microseconds to a 100 ms have been reported, and in general BBB opening is greater as the burst length is increased, with no real benefit observed after 10 ms (McDannold et al. 2008a, b; Choi et al. 2011, O’Reilly et al. 2010). The repetition frequency of the bursts has not significantly impacted the amount of BBB opening (McDannold et al. 2008b), however it is understood that if the burst repetition frequency is too high, the microbubbles cannot reperfuse the area, thereby limiting the effectiveness of the low ultrasound energy (McDannold et al. 2007; Yang et al. 2008; Goertz et al. 2010; Weng et al. 2010; Choi et al. 2010; Vlachos et al. 2011). The microbubbles themselves can impact BBB opening. As microbubble size and dose are increased, there is greater BBB opening and potential for damage (Samiotaki et al. 2012; Wang et al. 2014).
Until recently, FUS-mediated BBB opening was monitored by post-treatment magnetic resonance imaging (MRI) and histological methods; however these methods were limited in their ability to provide feedback during FUS treatments. Over the past few years, several different groups have developed methods to detect and evaluate microbubble activity in-vivo (McDannold et al. 2006; Tung et al. 2011; O’Reilly and Hynynen 2012, 2013; Arvanitis et al. 2012). It was demonstrated that the frequency content emitted from the microbubbles during the ultrasound treatment can be used for online control of the exposures (O’Reilly and Hynynen 2012).
16.5 Advantages of FUS for Drug Delivery Through the BBB
There are several advantages to FUS-induced BBB opening with microbubbles over other methods used.
Targeted
The ultrasound is focused so that the BBB is only opened in the targeted region of interest. The ultrasound is focused using one of two methods. Firstly, if using a single element transducer, as is common in preclinical studies, the surface of the transducer is curved so that the natural geometric focus comes to a point at a set distance from the transducer. Secondly, for a multi-element hemispherical transducer, which is commonly used for clinical applications, the elements have a geometric focus at the center of the sphere. To target areas away from the midline of the brain, the elements can be electronically steered to produce off-center focal points. These phased arrays are also needed for trans-skull focusing in humans since the variable thickness of the human skull distorts the propagating ultrasound wave, such that sharp focus is not achieved from a single element focused transducer (Hynynen and Jolesz 1998). The phased array allows precise control of the phase and the amplitude of the ultrasound wave emitted by each array element, thus providing a method for distortion correction and sharp, through skull focusing (Clement et al. 2001). By combining the phased array with MR imaging (Hynynen et al. 2004), targeting accuracy in order of 1 mm has been achieved when these focused beams have been used to coagulate deep human patient brain tissue (McDannold et al. 2010; Lipsman et al. 2013; Fig. 16.3).
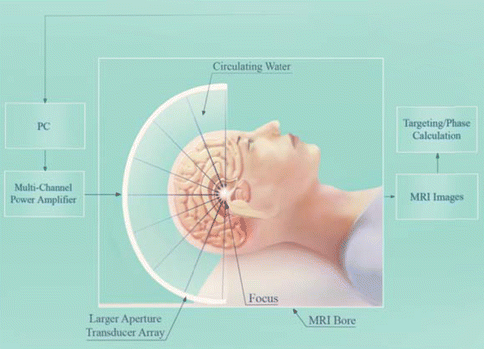
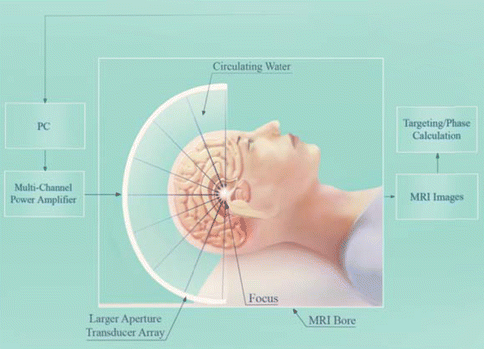
Fig. 16.3
For clinical application of FUS to open the BBB, a multi-element hemispherical ultrasound array is used. The elements can be steered electronically to target regions away from the midline. The ultrasound is combined with MR imaging to improve targeting accuracy to ~1 mm. These systems have been used to coagulate deep brain tissue in human patients
Some of the original methods used to open the BBB, including osmotic and chemical opening, produced widespread permeabilization of the brain and allowed potentially toxic components of the blood to access the entire nervous system (Rapoport 2001). Current and developing methods to overcome the BBB include modifying drugs so that they mimic antigens of endogenous brain endothelial receptors (Pardridge and Boado 2012). Although this method can be successful, drug delivery can be diffuse and it can be difficult to achieve therapeutic concentrations in the target region.
Transient
Following BBB opening with FUS, the BBB has been found to be closed when evaluated with a clinical MRI contrast agent dose approximately 6 h after opening. The BBB remained impenetrable for up to 4 weeks, the longest time point tested (Hynynen et al. 2001, 2006). The reversibility of BBB permeability is related to the extent of BBB opening. Therefore, the period of time before the BBB closes may be related to the pressure and pulse length of the ultrasound used to open the BBB (Samiotaki et al. 2012; Samiotaki and Konofagou 2013). There have been other reports that suggest that the BBB can remain open up to 24 h using similar pressure levels (Choi et al. 2007). Further research is required to fully understand the impact frequency, pulse length, acoustic pressure and the method used in determining the closure has on the reversibility of BBB permeability. However, it is not clear yet if the long term openings are also associated with some degree of tissue damage. This is given that detailed histological studies investigating ischemia, apoptosis and surviving neuron numbers have only been conducted at exposures that result in BBB closure approximately 6 h after opening, as determined by clinically applicable MRI methods (Hynynen et al. 2005, 2006).
Non-invasive
Ultrasound energy is capable of transmitting through the skull and brain tissue when appropriate frequencies and parameters are used. The non-invasive nature of this treatment eliminates the need for surgical procedures (Hynynen et al. 2001). In addition, the microbubbles that are required to create reproducible and safe BBB opening can be delivered intravenously.
Safe
When FUS is applied within an appropriate range of parameters and in conjunction with microbubbles, BBB opening can be achieved with no histological evidence of ischemia or apoptosis (Hynynen et al. 2005, 2006; McDannold et al. 2005). It has been suggested that BBB opening allows the entry of toxic blood components, including red blood cells and albumin. However, studies have shown that minor extravasations following transient, targeted BBB opening are cleared by glial cells, and have no effect on the neuronal populations (Alonso et al. 2011). Furthermore, cognitive and motor tests following repeated BBB opening with ultrasound have shown no adverse effects on behavior (McDannold et al. 2012). In a mouse model of Alzheimer’s disease, repeated application of FUS led to improved cognitive function and increased neurogenesis in the targeted brain areas (Burgess et al. 2014). Applying too much acoustic pressure may result in tissue damage (Hynynen et al. 2005), therefore the development of acoustic monitoring techniques to monitor and control the applied pressure will ensure a consistent and safe level of BBB opening (McDannold et al. 2006).
16.6 Mechanisms of FUS
FUS has proven to be an effective method for transiently opening the BBB and allowing therapeutic agents to cross into the brain parenchyma. Yet, the physical and cellular mechanisms by which the barrier is opened are still unknown. It has been shown that at low ultrasound pressures, microbubble oscillations put stress on the vascular endothelium (Hosseinkhah and Hynynen 2012), which responds to dynamic shear stress (Krizanac-Bengez et al. 2004). Although both the expansion and the contraction phases of the bubble under ultrasound can impact the blood vessel wall, recent evidence has indicated that the majority of changes occur during the contraction phase (Chen et al. 2011). Bubble contraction exerts forces that pull the vessel wall towards the interior of the lumen. There are also circumferential stresses and radiation forces that are generated by an oscillating microbubble that may activate the mechanosensitive ion channels in the vascular endothelium (Traub et al. 1999).
At higher pressure, microbubble oscillation can turn into violent collapse, a process known as inertial cavitation. It has been suggested that inertial cavitation could be a potential mechanism for BBB opening, but due to the extreme local temperature rise and microjetting, it is suspected that inertial cavitation is linked to red blood cell extravasation and more serious vessel damage (Nyborg 2001; Deng et al. 2004; Vykhodtseva et al. 2008). Recently developed methods, which monitor microbubble acoustic emissions during ultrasound exposure, have determined that BBB opening can occur in the absence of inertial cavitation. Analysis of the acoustic emissions demonstrated rises in subharmonics, harmonics and ultra harmonics due to bubble oscillation. These harmonic changes are correlated to BBB opening (McDannold et al. 2008b; O’Reilly and Hynynen 2012). The acoustic emissions observed during inertial cavitation show increased broadband emission, and these signals are correlated experimentally with red blood cell extravasation and damage, thus confirming that BBB opening is not due to inertial cavitation.
Less is known about the cellular mechanisms of FUS. Initial studies delivered labeled endogenous IgG and tracked its entry through the BBB following FUS in the presence of microbubbles (Sheikov et al. 2004, 2008). The studies suggested that antibodies passed through the BBB using both the paracellular and transcellular delivery routes.
Firstly, the paracellular space was increased in the FUS application region. This widening of the tight junctions was accompanied by a reduction in the quantity of tight junction proteins (Sheikov et al. 2004, 2008), suggesting increased paracellular transport following FUS application. Specifically, there was a reduction in key tight junction proteins (occludin, claudin-5 and zonula occludens) between 1 and 2 h after FUS application, but these levels were returned to normal within 4 h (Sheikov et al. 2004). These studies have been supported by research using oxidants to open the BBB, in which there was a consistent and similar reduction in occludin and zonula occludens (Musch et al. 2006). With respect to FUS, it has been hypothesized that FUS induces either protein reorganization in the tight junction which masks the antigens, or that the proteins are downregulated (Sheikov et al. 2008). It is known that FUS can downregulate other proteins, such as the gap junction protein Connexin 43 (Alonso et al. 2011). The change in gap junction proteins was suggested to buffer the hyperexcitability of the brain, which may occur after BBB opening (Alonso et al. 2011).
Secondly, there was labeled antibody present inside vesicles within the endothelial cells. Along with an increase in the number of cytoplasmic channels and vesicles in FUS-treated regions, it was evident that antibody was being taken up by the endothelial cells and passed through the BBB transcellularly (Sheikov et al. 2004, 2008). Related studies have demonstrated both in-vitro and in-vivo that caveolin, a membrane protein involved in receptor independent endocytosis, is upregulated after FUS (Lionetti et al. 2009; Deng et al. 2012). Uptake of the drug into the cells may occur during the deformation of the endothelial cells due to the shear stress generation by the microbubble in the ultrasound field (van Wamel et al. 2006; Meijering et al. 2009).
In-vivo, two-photon microscopy has been used to visualize FUS-mediated BBB opening. Two studies have attempted to fit the leakage observed during imaging sessions to the cellular mechanisms of FUS (Raymond et al. 2008; Cho et al. 2011). In these studies, fluorescent dye (10–70 kDa) was injected intravenously to visualize the vasculature and to observe the leakage kinetics as the dye passed through the BBB. Two different patterns of dye leakage from a single vessel were observed. Fast leakage was characterized by the dye rapidly crossing across the BBB within the first 5 min after FUS application, as well as appearing to arise from a point source along the vessel wall (Cho et al. 2011). Conversely, slow leakage began 5–15 min after the onset of FUS and occurred along the length of the vessel (Cho et al. 2011). The authors speculated that these leakage types corresponded to the different cellular mechanisms of FUS. Fast leakage was hypothesized to occur when tight junction proteins were downregulated, leading to tight junction widening and ‘fast’ leakage of dye through the BBB. Slow leakage was hypothesized to occur when the endothelial cells were stimulated to uptake dye and transfer it through the BBB. A next step will be to correlate two-photon microscopy with acoustic emission analysis to understand the relationship between the dye leakage type and the microbubble emissions.
16.7 FUS and Microbubbles for Drug Delivery
Over 100 primary research studies have used FUS to deliver tracers or therapeutic agents through the BBB and into the brain. Evaluation of BBB opening and quantification of drug delivery is done by delivery of a contrast agent or tracer molecule. Most commonly, gadolinium-based MRI contrast agents (500–900 Da) are delivered during FUS treatment, and the extravasation of these agents into the brain is used to confirm and evaluate BBB opening using MRI (Hynynen et al. 2001). MRI images are analyzed and the hyperintense regions, indicative of contrast agent delivery, are measured and used as an indicator of the ‘amount’ of BBB opening (Treat et al. 2007). It has been demonstrated that the relative enhancement of the hyperintense regions is correlated to the amount of vessel damage that occurs in the brain (Hynynen et al. 2001; McDannold et al. 2008a, b; O’Reilly and Hynynen 2012). Within the safe range of BBB opening (enhancement <30 %), there is the clear advantage of more drug delivery when the BBB is opened to a greater extent. This is particularly true when delivering large molecules. As an example, Jordao et al. measured the amount of endogenous IgM, an antibody that weighs 6 times more than the more abundant IgG. They demonstrated that the percentage increase in IgM in the brain after FUS-mediated BBB opening is positively correlated to relative amount of BBB opening, quantified by increased enhancement on MR images (Jordão et al. 2013). There is room for further experimentation in this area to precisely optimize the amount of BBB opening during each treatment with respect to the drug that is being delivered. It is understood that larger drugs require more BBB opening to reach therapeutic concentrations in the brain, and balancing optimal drug delivery with the risk of vessel damage will be important (Yang et al. 2011; Nhan et al. 2013; Chen and Konofagou 2014). Many other studies have supported these findings and have validated the use of MRI, not only as a method to choose the target location, but also as a method to evaluate drug delivery and success of the treatment (Kinoshita et al. 2006; Treat et al. 2007; Liu et al. 2010b; Aryal et al. 2013; Fan et al. 2013a). It is also possible to combine the therapeutic agents with MRI contrast agent molecules, and thus directly quantify the amount of drug delivered (Ting et al. 2012; Fan et al. 2013a, b).
16.7.1 Drug Delivery to the Brain in Rodent Models
Delivery of fluorescent and colorimetric dyes has provided knowledge on the localization of the BBB opening to the region of interest and relative quantity of potential drug delivery. Trypan blue and Evan’s blue (~70 kDa when bound to albumin in-vivo), horseradish peroxidase (40 kDa) and fluorescently tagged dextrans (3–2000 kDa) have all served as models of drug delivery (Sheikov et al. 2004; Raymond et al. 2007; Cho et al 2011; Choi et al 2010).
A major focus of FUS-mediated drug delivery has been on its application for brain tumors. Several types of chemotherapeutic agents have been delivered effectively to the brain in rodent models. Doxorubicin, a chemotherapeutic compound with widespread use, has limited success in the brain because of the BBB. In rat brain tumor models, FUS-mediated BBB opening has delivered therapeutic concentrations of doxorubicin to the tumor (Treat et al. 2007; Fan et al. 2013a, b). Repeated delivery of doxorubicin using FUS leads to significant increases in median survival times over animals treated with doxorubicin alone (Treat et al. 2012; Aryal et al. 2013). In addition to doxorubicin, methotrexate (Mei et al. 2009), 1,3-bis(2-chloroethyl)-1-nitrosourea (BCNU; Chen et al. 2010) and epirubicin (Liu et al. 2010a, b) have also been effectively delivered with FUS and show tumor-suppressing effects in the brain.
Antibodies represent another class of drugs effective for cancer treatment. Herceptin, a monoclonal antibody that targets the HER2/neu receptor found in 30 % of breast cancers, is effective against breast tumors. On the other hand, it is unable to treat brain metastases. It was first demonstrated by Kinoshita and colleagues that antibodies are able to be delivered through the BBB using FUS (Kinoshita et al. 2006), and ongoing treatment results in significant improvements in tumor reduction and survival time (Park et al. 2012). As a novel method to treat brain tumors expressing HER2/neu receptors, natural killer cells expressing a Her2 antigen were delivered to the brain using FUS (Alkins et al. 2013). The immune cells entered the brain in the brain tumor region in quantities that were sufficient for tumor reduction.
Although tumors have been a focus for drug delivery to the brain, FUS-mediated BBB opening has other additional applications. Several studies have suggested that FUS-mediated BBB opening may be an integral part of the treatment strategy for neurodegenerative disease. FUS and microbubbles were used to open the BBB in a transgenic mouse model of Alzheimer’s disease and their non-transgenic littermates. No quantifiable differences in the opening or closing behaviors were detected (Choi et al. 2008). With the knowledge that antibodies could be delivered through the BBB from the Herceptin study, two research groups used FUS to deliver amyloid antibodies into mouse models of Alzheimer’s disease (Raymond et al. 2008; Jordão et al. 2010). Amyloid antibodies were found bound to plaques in the regions treated with FUS and microbubbles (Raymond et al. 2008; Jordão et al. 2010). Furthermore, the antibodies delivered by FUS were found to significantly reduce mean plaque size and number after 4 days compared to control treated animals (Jordão et al. 2010).
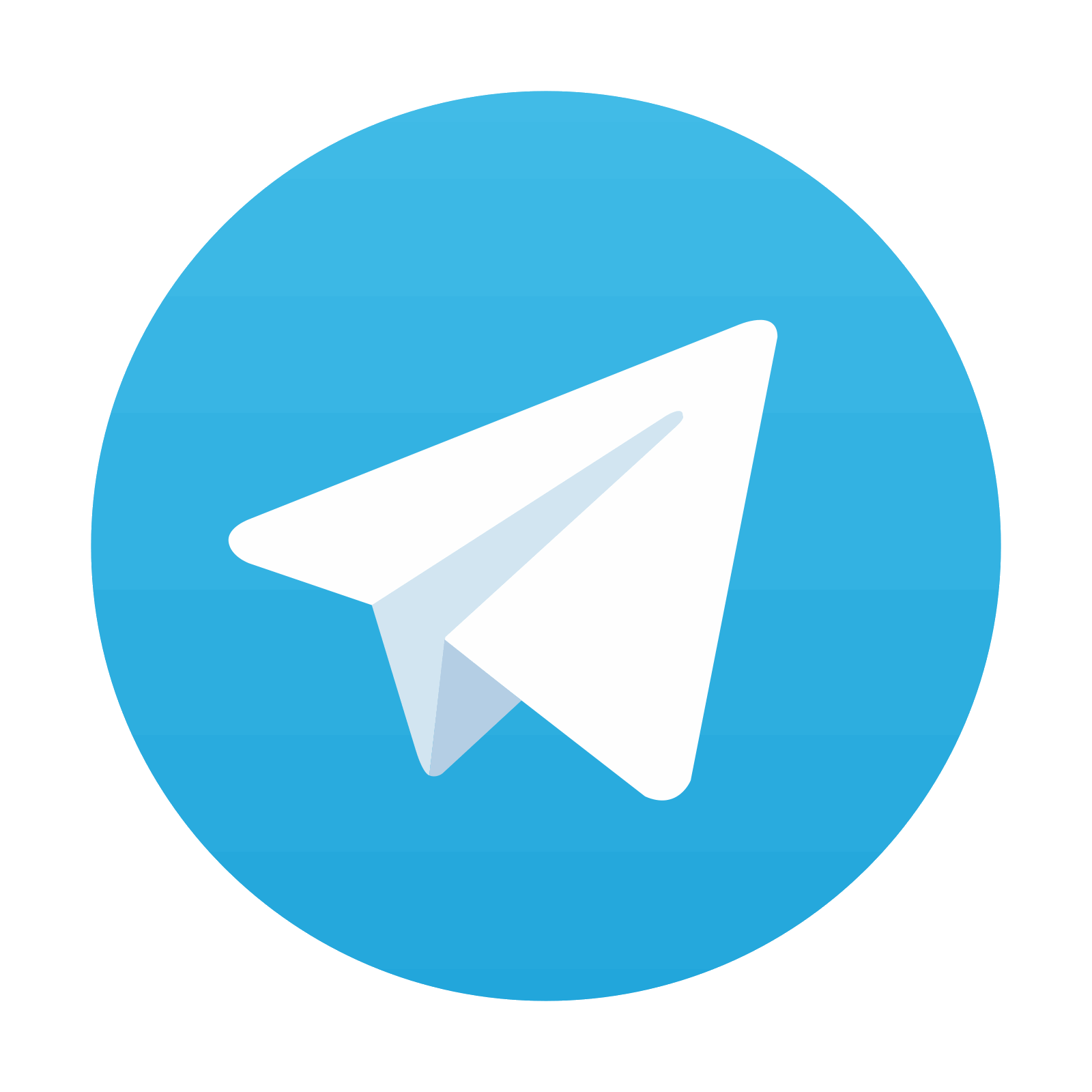
Stay updated, free articles. Join our Telegram channel
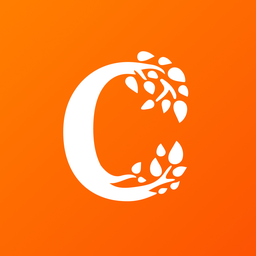
Full access? Get Clinical Tree
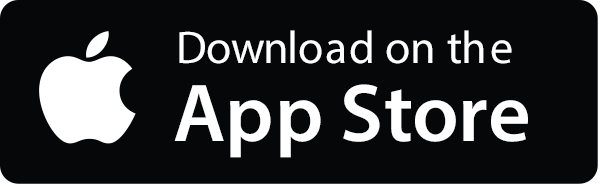
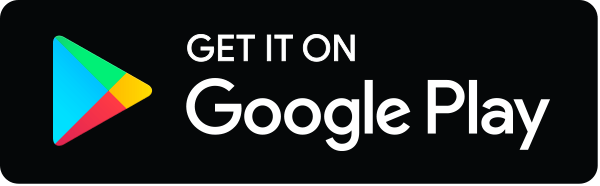