Fig. 1
A left frontal glioblastoma is shown in a conventional T1-w axial image (T1-w) without a contrast agent, in a map of quantitative R1 relaxation rate (qR1) with R1 = 1/T1, and in a map of proton density (PD). The qR1 map is gray scaled, showing the increasing T1 relaxation time from white to black; the PD map shows increasing PD values from black to white. The tumor is hypointense compared to white matter in the T1-w image due to the longer T1 relaxation time of tumor tissue (dark gray in qR1). However, the PD is increased in the tumor, increasing the signal in all weighted images of the standard MRI including T1-w images. This may attenuate the hypointense signal in T1-w images

Fig. 2
A glioblastoma of the splenium of the corpus callosum with adjacent subtle white matter changes which extended into both hemispheres and were suspicious for gliomatosis. Images from standard MRI (T1-w) and quantitative maps (qR1 and PD) are shown as in Fig. 1. T1 relaxation times of this infiltrative tumor are shorter compared to the glioblastoma in Fig. 1, resulting in a less hypointense appearance in qR1, while the PD is comparable. Thus, the tumor is hardly seen on standard T1-w images. Also note the bright signal in T1-w of the dorsal brain which is due to RF coil inhomogeneities. Quantitative mapping of theT1 relaxation time or its inverse, the R1 relaxation rate, eliminates the RF coil inhomogeneities and the PD contrast, thus showing the tumor much clearer in qR1. Even subtle changes of the adjacent white matter may be depicted
These ambiguous contrast phenomena can be avoided by acquiring a series of MR images with different T1 or T2 weighting which allows calculating the respective relaxation time. The resulting parameter maps provide quantitative values of the relaxation times (T1, T2) and thus offer more unequivocal information on tissue characteristics Schad et al. 1993. The following sections deal with the diagnostic value of these parameters.
However, the systematic approach to obtain these parameter maps is challenging. Current advances in MR imaging, hardware and post-processing techniques prepare the way to resolve these problems. The description of these quantitative methods is beyond the scope of this book and reference is made to some reviews (Cheng et al. 2012; Tofts 2003). Apart from their diagnostic value, these quantitative parameter maps are free from magnetic field inhomogeneities, RF coil characteristics and the specific choice of imaging parameters (e.g., TR, TE), thus allowing the quantification of pathological tissue changes. The diagnostic advantages of quantitative and semiquantitative MR parameter evaluation have been shown in MS patients (Cheng et al. 2012; Hasan et al. 2012; Tofts).
Unfortunately, most quantitative MR studies on brain tumors date from decades ago, i.e., the early stages of methodical development restricted by less developed hardware and low computational power. Damadian was the first to report prolonged T2- and T1-relaxation times in neoplastic tissue (Damadian 1971). Although the benefit of quantitative MR methods in differential diagnosis of brain tumors has yet to be shown, it should be considered as one of the most challenging responsibilities for radiologists to establish standardization and reliable MR parameter quantification in tumor imaging, especially in the context of tumor monitoring.
2 Transverse Relaxation Time T2
The spin-spin or transverse relaxation time T2 describes the exponential decay of the component of the tilted magnetization, which is perpendicular to the static magnetic field. Local inhomogeneities of the static magnetic field (e.g., due to magnetic susceptibility differences or chemical shifts) lead to an acceleration of this decay, which is described by the effective transverse relaxation time T2*.
The transverse relaxation rate (R2) experienced by a spin, which is the inverse of T2, depends on the energy transfer between two spins, yielding fluctuations of their respective Larmor frequencies. The T2 relaxation time is long in tissues with freely moving water molecules, such as those in pure water and aqueous solutions. Macromolecules (e.g., myelin, proteins) interacting with the water molecules influence the T2 relaxation time, which decreases as the concentration of macromolecules increases. Most brain pathologies (e.g., tumor infiltration, edema, gliosis) result in an accumulation of abnormal fluids and/or in a decrease of macromolecules within the brain tissue. Therefore, T2-weighted MR images (T2-weighted sequences in the strict sense and also FLAIR which displays a T1 contrast in addition to the T2 weighting) are used to detect brain pathologies as most of them appear bright against the darker normal tissue. Mapping of the relaxation time T2 in the brain quantifies changes of the normal and pathological tissue in an objective and reproducible manner.
Experimental animal studies as well as human studies demonstrated that glioma tissue has significantly longer T2 relaxation times compared to normal brain tissue (Englund et al. 1986; Hoehn-Berlage et al. 1992; Oh et al. 2005) and even compared to non-glial tumors (Englund et al. 1986; Oh et al. 2005). Positive correlation of T2 with ADC values in brain tumors implies that T2 values are related to the water content of the tumor. Therefore, higher T2 values indicate more necrotic tumors with lower tumor cell density, whereas a high cell density or a high amount of interstitial reticulin deposition lowers the T2 values of tumor tissue (Englund et al. 1986; Berghoff et al. 2012; Oh et al. 2005). Furthermore, a higher T2 relaxation time was observed in peritumoral edema as compared to normal tissue. It is well known from stereotactic surgery that the so-called peritumoral edema of gliomas is a mixture of vasogenic edema and tumor cell infiltration. The T2 value of the edema adjacent to the enhancing tumor (i.e., the tumor signal enhances in a T1-weighted image after application of a contrast agent) may help to differentiate metastases from gliomas: T2 values in the pure vasogenic edema of metastases and meningiomas were longer than those in the immediate peritumoral edema of gliomas (Oh et al. 2005). Despite these promising results, it should be emphasized that the characterization of neoplastic tissue changes such as necrosis, tumor cell accumulations, edema, and vasculature may not be determined by quantitative MRI alone, but also requires visual scrutiny of structural patterns.
This approach also helps to monitor tissue changes such as increasing tumor infiltration over time by using subtraction maps. For this purpose, consecutive follow-up maps of the relaxation time T2 of an individual patient are co-registered and subtracted voxel-wise from a reference map of the same patient. This allows the detection even of subtle tumor infiltration which might not be detectable by visual inspection (Hattingen et al. 2013).
Apart from tumor infiltration, acute therapeutic reactions like radiation necrosis may also increase the T2 relaxation time (Larocque et al. 2009). However, T2 values can also decrease under radiation, possibly due to the presence of paramagnetic substances such as blood products. Our own experience from T2 mapping of glioblastomas is that differences in T2 relaxation times are highest between edema and normal brain tissue, whereas the tumor tissue demonstrates a wider range of T2 values sometimes even similar to normal brain tissue. In particular, antiangiogenic therapy of patients with progressive glioblastomas resulted in a significant decrease of the T2 value in tumor tissue approaching T2 values similar to normal brain tissue (Hattingen et al. 2013). Tumor tissue with a low T2 relaxation time exhibits a reduced signal on T2-weighted images, reducing the perceptibility of these “darker” tumors which might thus be visually missed. This could explain why progression-free survival – but not the overall survival – is longer under antiangiogenic therapy compared to other treatment modalities: real progression of non-enhancing tumors may be visually missed under antiangiogenic therapy. The lower T2 values seem to reflect a normalization of the blood-brain barrier (BBB) under antiangiogenic therapy, which reduces edema and therefore tumor water content.
In summary, T2 relaxation time mapping of brain tumors seems to be sensitive to the detection of tumor-related tissue changes and might be an excellent tool for longitudinal tumor monitoring (T2 difference maps), whereas the specificity of the changes is limited.
3 Effective Transverse Relaxation Time T2* and Susceptibility-Weighted Imaging (SWI)
The effective transverse relaxation time T2* reflects the dephasing of the transverse magnetization in gradient echo sequences which is due to the spin-spin relaxation and local field inhomogeneities. Therefore, T2* is influenced by local microscopic and macroscopic magnetic field inhomogeneities due to susceptibility differences between tissue types and chemical shifts. Macroscopic field inhomogeneities (B0) are found near the skull base and may also be induced by metallic devices. Microscopic field inhomogeneities result from physiological iron depositions in the deep nuclei of the brain and deoxyhemoglobin (deoxyHb) in blood. The relation of diamagnetic oxyHb to paramagnetic deoxyHb mainly influences the T2* relaxation time in brain tissue, which is also known as “blood oxygenation level-dependent” (BOLD) effect. In summary, T2* is influenced both by spin-spin relaxation (which is described by T2) and susceptibility effects (which are described by the relaxation time T2′). The mathematical correlation of these three relaxation times is 1/T2′ = 1/T2* − 1/T2 or alternatively when using the relaxivities R′ = R2* − R2. Measuring T2* and T2 relaxation times, B0 field inhomogeneities and blood volume fraction yield reliable information on the oxygenation of brain tumors (Fig. 3), so that T2′ mapping in combination with an MR perfusion measurement may be considered as “hypoxia imaging” (Tóth et al. 2013). Tumor hypoxia is considered as an important motor of malignant transformation and, therefore, increases the tumor’s aggressiveness. It also causes resistance of the tumor to chemotherapy and radiation mediated by the activation of HIF-1α and carbonic anhydrase IX and XII (Harguindey et al. 2009). The indirect measurement of hypoxia by T2′ quantification might provide insight into the pathophysiology of brain tumors and into the effects of therapy (Hattingen et al. 2013; Hoskin et al. 2007). Saitta et al. could show that high-grade tumors revealed lower T2′ values, suggesting a higher degree of hypoxia in these fast growing and proliferating tumors (Saitta et al. 2011). However, it is not possible to differentiate deoxyHb from other microscopic sources of susceptibility effects like tumor microbleeds. This might result in confounding results in glioblastomas, since microbleeds are a very typical characteristic of this type of brain tumor. It has turned out that the detection of microbleeds in high-grade gliomas is very helpful for discriminating them from other space-occupying pathologies such as demyelinating lesions, lymphomas, and metastases (see below).


Fig. 3
Compilation of different parameter maps from quantitative MRI in a patient with left frontal glioblastoma: the upper row shows maps of the transverse relaxation times T2, T2*, and T2′ (qT2, qT2*, and qT2′) and a conventional T2-weighted image (T2-w); the lower row shows maps of the longitudinal relaxation time before (qT1) and after application of a contrast agent (qT1+CA), the difference map (Diff) resulting from voxel-wise subtraction of (qT1+CA) from qT1, and a conventional T1-weighted image after application of a contrast agent (T1w+CA). The standard MR images (T2-w and T1w+CA) show the tumor’s central necrosis, which is surrounded by solid tumor tissue enhancing after CA application, and a large edema. The images also show that the non-enhancing part of the tumor infiltrates the corpus callosum and the basal ganglia and that there is a small area of edema in the contralateral frontal lobe. However, the maps of the different relaxation times reveal further biological information: The T2′ map (which is T2* without T2 effects) reveals some dark areas which might represent hypoxia and/or tumor bleedings (white stars). The qT1 map depicts the whole tumor extension and its relation to the anatomical structures showing high gray-white matter contrast (please note the inverse contrast compared to the qR1 map in Figs. 1 and 2; in qT1 the T1 relaxation time increases from black to white). The CA shortens the T1 relaxation time (dark areas in qT1+CA). However, voxel-wise subtraction of qT1+CA from qT1 yields a T1 difference map (Diff) additionally depicting tumor enhancement in the corpus callosum and basal ganglia which is missed on T1-weigthed images after application of a CA (T1w+CA) (white arrow heads). Additional slight enhancement is also seen in the “edema” region (white arrows), suggesting angiogenetic reactions from invisible tumor infiltrations
Since microbleeds are best detected with high-resolution susceptibility-weighted imaging (SWI), this method plays an important role in tumor diagnosis. Since field inhomogeneities and thus susceptibility effects increase with the field strength, this relatively new MR technique benefits from higher field strengths which become increasingly available in the clinic. In SWI, filtered phase and magnitude information are combined to create a new susceptibility-weighted image contrast (Haacke et al. 2004). SWI visualizes normal or pathological venous structures and microbleeds that are not visible on conventional MR images. Pronounced intratumoral susceptibility signals (ITSS) are found in almost all glioblastomas but not in CNS lymphomas, demyelinating diseases, and low-grade gliomas (Mittal et al. 2009; Park et al. 2010; Peters et al. 2012). In most cases, microbleeds in metastases are less pronounced compared to microbleeds in glioblastomas (Park et al. 2010). Furthermore, intralesional venous structures differ considerably between different space-occupying brain lesions. The perivenous inflammation in multiple sclerosis (MS) lesions shows a typical SWI pattern revealing normal venous structures which are the lead structures for the MS plaques (Mittal et al. 2009). In contrast, high-grade gliomas not only show prominent microbleeds, but also a distorted and irregular neovasculature (Kim et al. 2009; Mittal et al. 2009).
4 Longitudinal Relaxation Time T1
Several studies showed a positive correlation between the brain water content and the T1 relaxation time (MacDonald et al. 1986; Fatouros et al. 1991). The positive correlation of the mean diffusivity of water (Bastin 2002) and the T1 relaxation time and a significant T1 reduction under antiedematous therapy support the hypothesis that the T1 relaxation time reflects the tissue water content (Andersen et al. 1993). Longer T1 values were found in enhancing lesions of MS patients compared to non-enhancing lesions, indicating a disruption of the BBB and hence a higher water content in the adjacent brain tissue prolonging T1 (Jurcoane et al. 2013). Human and animal studies showed prolonged T1 relaxation times in glioblastomas and their peritumoral edema compared to normal brain tissue (Englund et al. 1986; Hoehn-Berlage and Bockhorst 1994) and other tumors such as meningiomas and schwannomas. In contrast, necrotic tumor tissue might display a shortened T1 relaxation time which might be due to the presence of methemoglobin or mineralization (Bähr et al. 2011; Boyko et al. 1992). It should be mentioned that T1 shortening might also be a prognostic marker for the survival time of patients with recurrent glioblastomas under antiangiogenic therapy (Bähr et al. 2011, 2014).
The presence of contrast agents containing gadolinium (Gd) or manganese (Mn) shortens the T1 relaxation time considerably. Furthermore, co-registered maps of the longitudinal relaxation time before (qT1) and after application of a contrast agent (qT1+CA) allow for voxel-wise subtraction of (qT1+CA) from qT1 (Fig. 3). This approach enables the quantification of an even subtle contrast agent enhancement independent of visual detectability (Jurcoane et al. 2013), yielding sensitive quantification of the BBB damage (Jurcoane et al. 2013).
However, further studies are required to answer the question if T1 mapping, alone or in combination with T2 mapping, histograms of T1 and T2 relaxation times in the tumor tissue and edema, and more sophisticated three-dimensional tumor segmentation methods are valuable tools to delineate tumor tissue and edema and if these techniques are of help in differential diagnosis and therapy monitoring.
5 Chemical Exchange Saturation Transfer (CEST)
The CEST method is based on the magnetic transfer effects of specific protons in metabolites (and exogenous contrast agents) that are in chemical exchange with the solvent. The method for detecting CEST contrast is similar to the magnetization transfer (MT) contrast, i.e., the intensity change of the water signal is monitored in the presence of off-resonance saturation. A CEST effect is observed when the off-resonance frequency matches the MR frequency of a metabolite proton which is exchanging with the water protons at an appropriate rate. By varying the frequency, power, and timing of the saturation pulses, CEST contrast can be tuned to signals from endogenous mobile proteins and peptides (amide proton transfer, APT) (Zhou et al. 2003a, b) to amino protons of glutamate (gluCEST) (Cai et al. 2012) or to hydroxyl protons like those from glucose (glucoCEST) (Chan et al. 2012; Nasrallah et al. 2013). Since the basic principle of the contrast relies on proton exchange with the solvent and therefore depends on the concentration of solvent protons, the method allows to measure tissue pH (Zhou et al. 2003b).
6 Cest Method
There are several articles which review the basic principles of CEST (Sherry and Woods 2008; van Zijl and Yadav 2011; van Zijl et al. 2003). Briefly, the method exploits the magnetization transfer (MT) effect which is well known from MT-MRI (Tofts 2003). In MTR contrast is generated by selectively irradiating off-resonance from the water signal, modifying the magnetization of macromolecules which are in contact with the water. By several mechanisms, this magnetization is transferred to the water protons detected with conventional MRI, causing a partial saturation and consequently a signal modification. The efficiency of the magnetization transfer is typically changed in several pathological situations providing a surrogate marker for affected tissues (Tofts 2003). Like in MTR, CEST-MRI monitors the effect (signal reduction) of an off-resonance irradiation on the water signal; however, the target molecules for irradiation are rather small (amino acids, peptides, glucose, exogenous agents with specific properties) and have a well-defined typical resonance frequency which is known from MR spectroscopy (see Chap. MR Spectroscopic Imaging). Some of the protons in these molecules are exchanged with the solvent (water). Selective saturation of these protons will transfer their magnetization to the MRI-detectable water signal, enabling the quantification of the exchange partner by measuring the signal changes of the solvent.
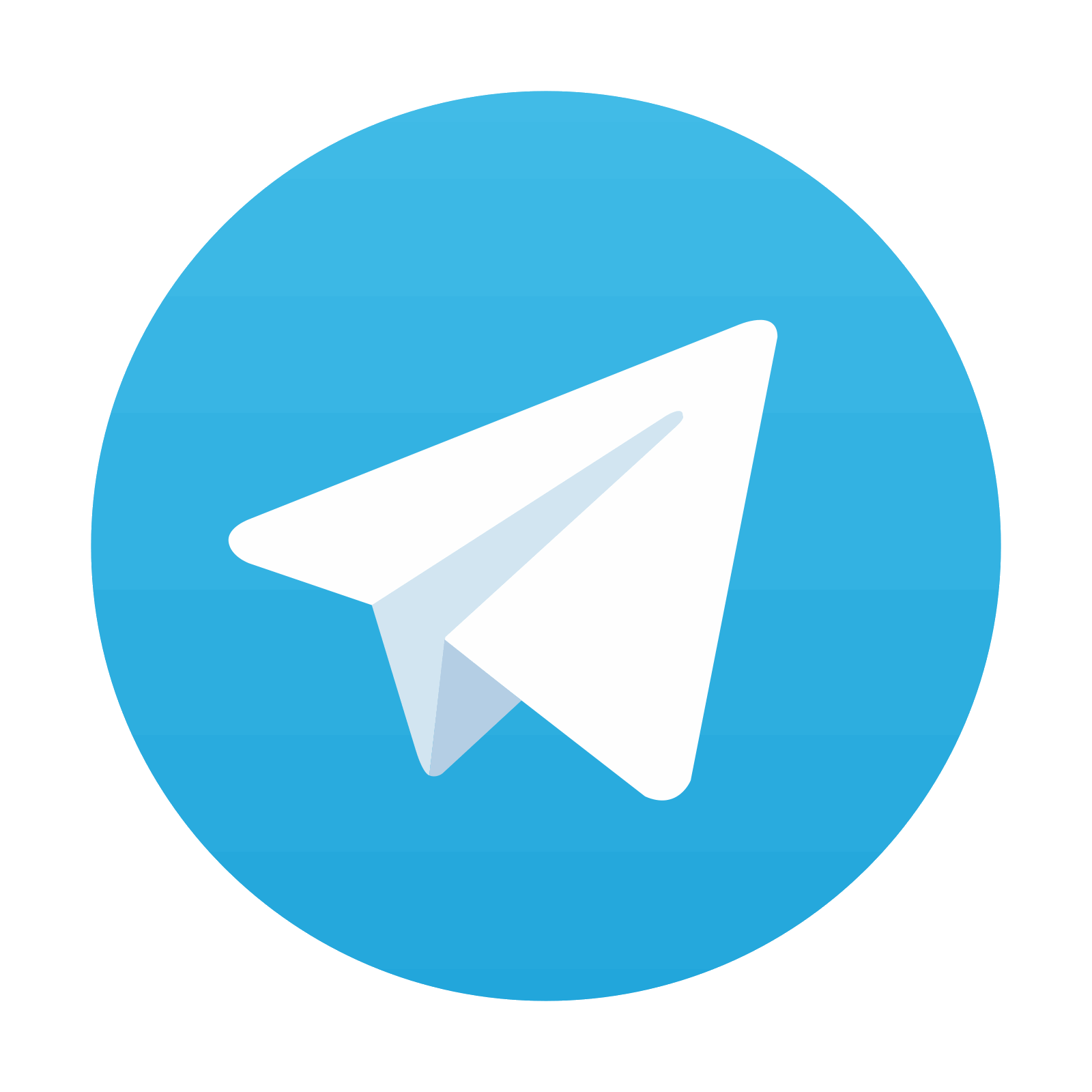
Stay updated, free articles. Join our Telegram channel
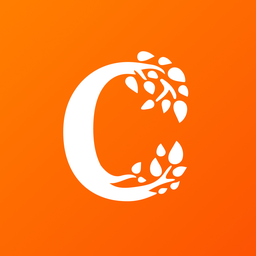
Full access? Get Clinical Tree
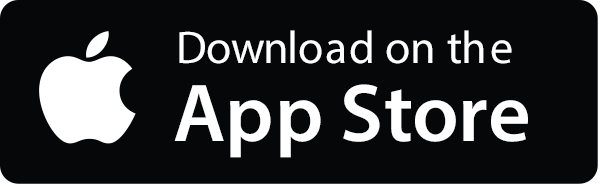
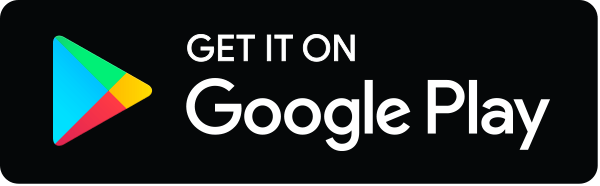