Fig. 1.1
The first recorded image of an infant, published in the Archives of Clinical Skiagraphy in May 1896. Note the apparent situs inversus (no markers to indicate side)
Imaging, of course, is not without risk. In 1904, Clarence Dally, Thomas Edison’s x-ray assistant, died from metastatic radiation-induced carcinoma after having radiation burns on his face, ulcers on his hands, and both arms amputated [2]. Although there is currently no increased incidence of cancer mortality among radiologists, the incidence was as high as 41 % for the earliest radiologists [3]. However, increasing evidence suggests that low-level radiation may cause fatal cancer [4, 5]. In 2005, the National Institute of Environmental Health Sciences formally added x-rays and gamma rays to the list of known carcinogens.
The overriding goal of imaging is to provide a timely and accurate diagnosis while limiting risks and controlling costs. Strategizing the appropriate imaging choice should utilize evidence-based medicine, usually starting with radiographs before moving to complex studies. This chapter discusses how clinical assessment affects imaging choices and introduces appropriateness criteria that can help determine the “best” imaging study. It reviews the strengths and weaknesses as well as costs, benefits, and risks of different imaging techniques. It also presents a brief review of technical factors that affect image quality and radiation exposure.
1 Assessing the Patient
1.1 Clinical Evaluation
Clinical evaluation, including history, physical examination, and laboratory investigation, is paramount to any imaging strategy. The history, beginning with the patient’s age, assesses the character of symptoms, their chronicity, and whether there has been trauma (although, especially in children, lack of trauma history does not necessarily exclude trauma). Physical examination may indicate point tenderness or the presence of regional or generalized pathology; there may be signs of inflammation or complex findings of congenital abnormalities. Laboratory investigation can help differentiate infectious/inflammatory conditions, malignancy, and systemic disease.
The radiographic evaluation of a limping infant or child, a common reason for imaging, illustrates how clinical information affects the imaging evaluation [6, 7]. The history may indicate an acute condition, such as a fracture, or a chronic condition, such as developmental dysplasia of the hip (DDH). The specific gait abnormality and the point of maximum tenderness can help focus the radiographic workup. For instance, if a toddler acutely stops walking and there are no signs of infection, then a toddler’s fracture is likely and radiographs of the tibia and fibula are indicated [8]. If this study is negative, imaging the pelvis and remainder of the affected limb may be appropriate. Limited motion, fever, and elevated inflammatory markers (white blood cell count, C-reactive protein, and erythrocyte sediment rate) suggest infection or inflammation that may require further workup to evaluate for septic joint or osteomyelitis.
1.2 Importance of the Point of Maximum Tenderness
Knowledge of the point of maximum tenderness is essential. This both allows the clinician to order the appropriate imaging study and helps the radiologist deliver a correct interpretation. In a large series of diagnostic errors from a United Kingdom emergency department, 5.4 % of fractures and 32 % of dislocations were missed because the wrong radiographs had been ordered [9].
Knowledge of the point of maximum tenderness helps the clinician order the appropriate imaging study in the setting of acute trauma. A strict imaging protocol can eliminate 12 % of upper extremity and 19 % of lower extremity radiographs, missing only 1 fracture out of 287, with significant savings in costs [10]. If the Ottawa ankle rules (OAR) are applied on children older than 5 years of age, a meta-analysis of 12 studies found a fracture detection sensitivity of 98.5 % (missing 10 out of 671 fractures), while 24.8 % of the radiographs could have been eliminated [11]. A smaller multicenter study validating the Ottawa knee rules in children reduced knee radiographs by 31.2 % without missing a single fracture [12]. Applying clinical evaluation criteria to trauma patients can eliminate unnecessary radiation and save costs. Though it takes time to assess patients for these protocols, it is better for the patient and for healthcare.
Understandably, knowing the location of maximum tenderness allows for more focused and intense evaluation of the site of injury. Radiologists improved their fracture detection rate by 6 % when they learned the location of pain [13]. Orthopedic surgeons’ fracture detection improved by 11 % [14] (Fig. 1.2).
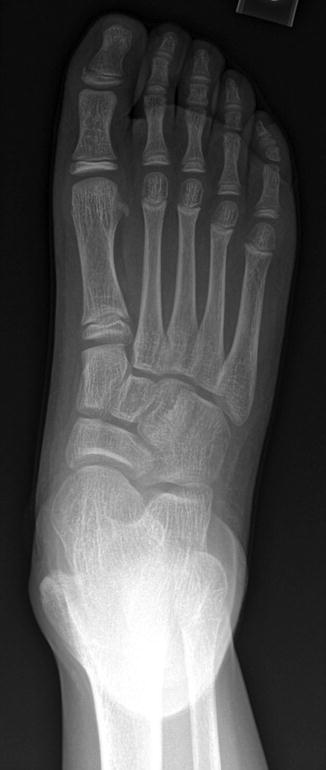
Fig. 1.2
Importance of knowledge of the point of maximal tenderness. There are subtle metaphyseal buckle fractures of the distal third and fourth metacarpals in a 12-year-old boy. However, the history did not identify the point of maximal tenderness, and the fractures were not recognized at the outside institution. One week later, the child was referred to our orthopedic clinic, where the same image was reviewed, this time with knowledge of the location of maximal tenderness. The fractures were now correctly identified
A small percentage of acute fractures will be radiographically occult. Therefore, if a fracture is strongly suspected yet radiographs are normal, the patient’s injury should be immobilized and imaged again 2 weeks later, by which time radiographs should show evidence of a healing fracture, such as resorption along the fracture line and development of periosteal reaction [15].
1.3 Imaging Acute Trauma in the Emergency Room Setting
Of all emergency imaging requests, those for skeletal trauma are probably the most common. It is estimated that over 50 % of emergency department visits are for minor injuries, many of which require radiographs. Radiographs help triage patients and sometimes direct further workup. It has been found that triage nurses can expedite visits and improve patient satisfaction by ordering radiographs before a physician sees the patient. Furthermore, radiographs ordered by appropriately trained triage nurses demonstrate fractures at a significantly higher rate than those ordered by senior house officers [16].
Imaging in the setting of acute blunt trauma is controversial but is frequently driven by hospital-based protocols. Protocols and literature-based evidence sometimes disagree, leading to over (or under) utilization of imaging. Many centers routinely obtain radiographs of the cervical spine, chest, and pelvis regardless of the clinical situation. However, the National Emergency X-Radiography Utilization Study (NEXUS) evaluated pediatric cervical spine injury to identify candidates for imaging studies [17]; they suggest that in the absence of midline cervical tenderness, intoxication, altered level of alertness, focal neurologic deficit, or distracting painful injury, there were no cervical spine injuries; 20 % of imaging studies would be eliminated if these criteria were applied. The Canadian cervical spine rules simplify the algorithm for determining the need for imaging the cervical spine. This system is based on assessing high-risk factors (age, dangerous mechanism, or paresthesia), low-risk factors that allow for safe assessment of range of motion, and the patient’s ability to rotate the neck 45° to the right and left. Almost half of the radiographs in a study population could have been eliminated by following these rules [18]. There is, however, much debate about applying NEXUS or Canadian cervical spine rules, especially for those under 10 years of age [19].
Similarly, pelvic radiographs may be ordered more often than necessary in the setting of blunt trauma. A study evaluating clinical correlates with pelvic fracture found no pelvic fractures if a child had not injured the lower extremity, had a normal physical examination of the pelvis, and had no indication for an abdomen/pelvic CT [20]. In another study of pelvic trauma, if there was no high-risk mechanism of injury, high-risk clinical findings, or pelvic tenderness, and if the Glasgow Coma Scale was ≥14, fracture could be excluded in 152/504 trauma patients, and pelvic radiographs could have been eliminated in 30 % of patients [21]. Multicenter prospective trials are needed to optimize blunt trauma imaging protocols in children.
2 Choices of Imaging Technique
2.1 Radiography
Imaging of the pediatric patient usually starts with radiographs, which are readily available, inexpensive, deliver minimal radiation, and often answer the clinical question [8, 22–28]. Even for soft tissue masses, radiography can help identify calcification, bony erosion or disruption, and foreign bodies [28].
Radiography is often sufficient in the acute setting of trauma [15]. The point of maximum tenderness should be imaged in at least two orthogonal planes, with three views for such complex areas as the foot/ankle and hand/wrist.
Routine contralateral imaging is generally unnecessary [29, 30], but in select cases, imaging the opposite side for comparison can help diagnose occult fractures, including subtle plastic bending fractures, hairline fractures, impaction fractures, Salter–Harris fractures, and buckle fractures [31]. Opposite side imaging is usually performed in pelvic radiography to assess femoral head ossification and acetabular development [29]. In unique cases, comparison views may avoid more expensive imaging tests such as CT and MRI (Fig. 1.3).
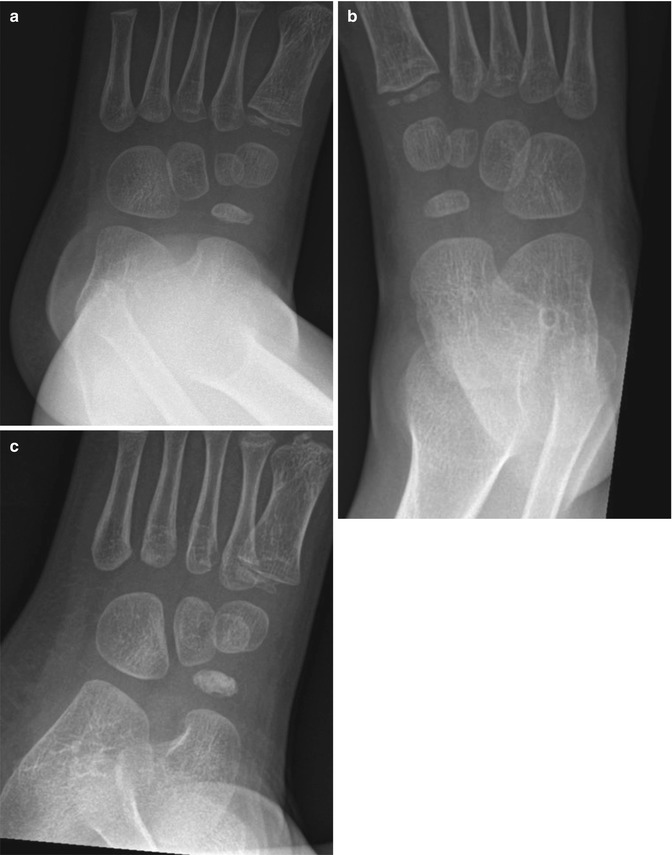
Fig. 1.3
Use of selected comparison view. Kohler disease in a 3-year-old girl with pain at the left mid-foot. The patient had been limping for 4 weeks and had no history of trauma or signs of infection. (a) Initial radiograph of the left foot shows subtle flattening of the tarsal navicular bone, more obvious when compared with (b), a radiograph of the right foot obtained at the same time. (c) Three weeks after supportive treatment in an orthopedic shoe, oblique view of the left foot shows patchy sclerosis, denoting early healing. In this case, initial correlation with the normal contralateral side confirmed that the tarsal navicular on the left was indeed abnormal and also allowed confident diagnosis. While not routinely performed, selective opposite extremity views can help establish the diagnosis and eliminate the need for complex imaging such as MRI (expensive, with attendant sedation risks in a 3-year-old) or nuclear medicine bone scan (radiation risk and costs)
2.2 Ultrasound
Limited research suggests that US can detect radiographically occult fractures with high sensitivity and specificity (Fig. 1.4), but it is uncertain to what extent detection of these occult fractures alters management [32].
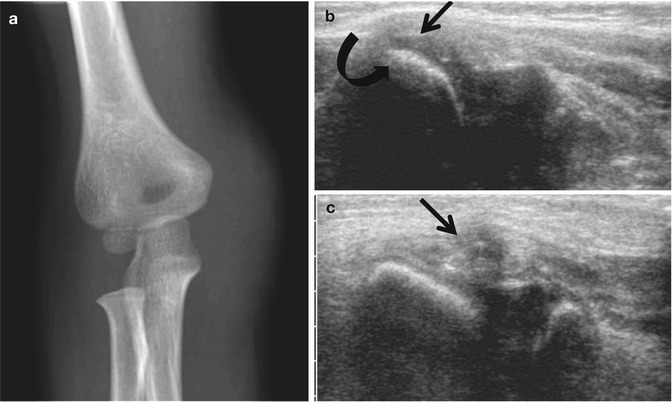
Fig. 1.4
Use of US to identify cartilaginous fracture in a 4-year-old who landed on the right elbow after jumping off a couch. (a) Frontal elbow radiograph shows soft tissue swelling over the non-ossified medial epicondyle. (b) Longitudinal US of the normal left medial epicondyle (for comparison) shows the normal relationship of the hypoechoic medial epicondyle (arrow) to the echogenic medial condylar cortex (curved arrow). (c) Comparable US of right medial epicondyle demonstrates fractured cartilage (arrow)
In some situations, radiographs are not appropriate for initial imaging. For example, US is recommended to evaluate for DDH in infants less than 6 months old. US stratifies patients best, reduces the number of patients in Pavlik harness, does not increase the incidence of “missed” DDH at age 2 years, and does not increase costs [33].
US is also the appropriate second imaging test (after radiographs) for most soft tissue and joint issues, as it is relatively inexpensive and does not involve ionizing radiation or sedation [28]. US readily detects radiolucent foreign bodies, classifies soft tissue masses as cystic or solid, and characterizes vascular flow. The main limitations to US include limited operator and interpreter expertise, long learning curve, and resistance on the part of referring physicians (as US images are difficult to understand without experience). Furthermore, although US can evaluate bone cortex for abnormalities such as fractures, it is otherwise limited in its ability to assess bones. It is employed in adults to assess tendon/ligament pathology and rheumatologic diseases of the shoulder, elbow, and wrist. Although its use is less widespread in children, increased concern about radiation exposure has led investigators to study US to evaluate congenital and developmental anomalies, ligament injury, and arthropathies.
2.3 Computed Tomography
CT is in general readily available and rapidly performed; multiplanar isotropic voxel imaging allows reconstruction in any plane at submillimeter thickness. It is superb for evaluation of cortical bone, periosteal reaction, and soft tissue calcification. CT provides excellent anatomic detail in evaluation of acute trauma, especially for complex pelvic fractures or Salter–Harris injuries of the knee or ankle. Contrast CT angiography is as effective as angiography for assessing vascular extremity injury in acute trauma [34]. CT accurately assesses biological response of osteosarcoma to chemotherapy [35], but in general MRI surpasses CT in evaluating soft tissues and bone marrow in patients with suspected infection and tumor. High radiation exposure and cost are CT’s main drawbacks.
2.4 Magnetic Resonance Imaging
MRI has revolutionized musculoskeletal imaging. Multiplanar imaging is routinely performed without ionizing radiation. MRI excels at evaluating muscle, soft tissue, and bone marrow pathology because of its exquisite detail and tissue contrast. Sequences that accentuate differences in T1 and T2 relaxation times between normal and pathological tissues help characterize lesions. Contrast is rarely needed in post-traumatic evaluation of muscle, tendon, cartilage, and bone. MRI arthrography (MRA) may help evaluate labral injuries of the hip and shoulder. Gadolinium-based intravenous contrast agents help assess tumors as well as infection/inflammation and abscesses. Whole-body MRI techniques that evaluate bone marrow are becoming more common [36]. While MRI has superior sensitivity to bone scintigraphy in the detection of skeletal metastases, it has significantly decreased sensitivity to small extraskeletal metastases, especially in the lung and liver [37].
However, MRI is expensive and may require sedation or general anesthesia. Nephrogenic systemic fibrosis develops after gadolinium administration in some patients with renal insufficiency, and, although there are few reports of this in children [38], this has led to more cautious application of contrast material in all age groups. Metallic implants may cause localized artifact. Furthermore, both known and unknown metallic material within the patient can move, with potentially disastrous results (MRI safety of different devices can be reviewed at http://www.mrisafety.com/default.asp or by contacting the device manufacturer).
2.5 Nuclear Medicine Imaging
Nuclear medicine imaging (NM) contributes to evaluation of many orthopedic disorders, including tumors, occult trauma, child abuse, and osteomyelitis/inflammation. Bone scans are sensitive at detecting lesions in the child with subacute or chronic nonlocalizable musculoskeletal pain. Positron emission tomography (PET) and PET/CT are important for oncologic imaging [39]. Recent research suggests that sodium fluoride (18F-NaF) has higher spatial resolution and performs better than technetium-99m methylene diphosphonate (Tc-MDP) bone scan at a comparable dose, such as in the setting of child abuse [40], but currently it is not widely used. Bone scintigraphy, like CT, involves relatively high radiation exposure [39].
3 Cost and Optimizing Imaging Selection
Decisions about which study to perform should be based on the most cost-effective test that answers the clinical question. Two-thirds of referring physicians rely on advice from radiologists when selecting imaging tests [41]. The American College of Radiology (ACR) has developed evidence-based guidelines to help determine the best imaging test [8, 22–28]. However, only 30 % of radiologists use the musculoskeletal appropriateness criteria in their practice [42], and few referring physicians are aware of or employ these criteria. When general practitioners employ appropriateness criteria in ordering MRI’s, the studies are more likely to be approved at insurance review [43].
In a survey of high-litigation risk specialists (emergency medicine, general surgery, orthopedic surgery, neurosurgery, and obstetrics/gynecology), over 50 % practice defensive medicine by ordering more tests than are medically indicated [44]. Seventy-six percent of emergency medicine physicians order imaging tests that are not medically indicated because of malpractice concerns. These tests, of course, increase costs as well as radiation exposure.
Medicare musculoskeletal imaging costs in the USA in 2010 were estimated to be $969 million for MRI and $851 million for radiographs. By 2020, these costs are projected to increase to $2,046 million for MRI and $1,266 million for radiographs. By 2020, $736 million per year could be saved by substituting US for MRI when appropriate [45]. The 2005 Deficit Reduction Act was enacted to reduce imaging payments to control Medicare costs. This Act attempted to decelerate the growth of imaging examinations, but the number of tests continues to increase, particularly MRIs performed by non-radiologists in the office setting [46]. Cost containment can be achieved when ordering imaging studies in pediatric patients by using objective evidence-based imaging strategy (such as the OAR) along with appropriateness criteria.
4 Radiation Exposure
Between 1987 and 2006, the average yearly total radiation a person living in the USA received nearly doubled, from 3.6 millisievert (mSv) to 6.2 mSv [47]. This increase was due to medical imaging. In 1987, medical radiation accounted for 15 % of the annual radiation (0.53 mSv); in 2006 it accounted for 48 % (3.0 mSv) (Fig. 1.5). CT currently accounts for nearly 25 % of annual background radiation exposure (Fig. 1.6).
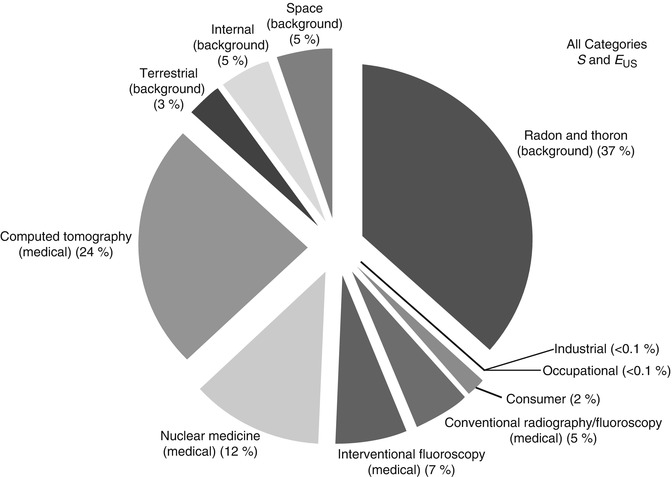
Fig. 1.5
Contribution of different forms of background radiation and different forms of medical radiation to overall radiation exposure in 2006. The total effective dose per individual was 6.2 mSv (whereas it was 3.6 mSv in the early 1980s). In 2006, medical radiation accounted for 48 % of the effective dose, whereas in the early 1980s it accounted for only 15 %. (Percent values have been rounded to the nearest 1 %, except for those less than 1 %.) (Reprinted from Ref. [48], with permission of the National Council on Radiation Protection and Measurements, http://NCRPpublications.org)
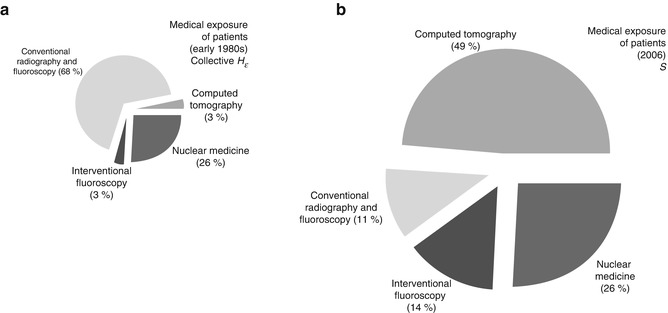
Fig. 1.6
Comparison of collective doses for computed tomography, conventional radiography and fluoroscopy, interventional fluoroscopy, and nuclear medicine (as percent of total collective dose) as reported for the early 1980s (a) and for 2006 (b). The percentage of effective dose from conventional radiograph and fluoroscopy decreased from 68 % to 11 %, while CT increased from 3 % to 49 % and interventional fluoroscopy increased from 3 % to 14 %. Note the disproportionate size of the pie charts reflecting the nearly 6-fold increase in medical radiation in the intervening years (Data from National Council on Radiation Protection Report 160. Reprinted from Ref. [48], with permission of the National Council on Radiation Protection and Measurements, http://NCRPpublications.org)
The International System (SI) terms describe radiation exposure: the two most important metrics are gray (Gy) and sievert (Sv). Gray is the energy imparted to a tissue in joules/kilogram. One Gy is equal to 100 rads. The Gy is frequently used to indicate the absorbed organ dose. Sievert is the equivalent or effective dose. The Sv is typically used to equate an imaging test that covers a limited area, such as a chest CT, to uniform, whole-body irradiation. One Sv equals 100 rem. Because some organs are more susceptible to radiation, the Sv is calculated by multiplying the organ dose in Gy by a tissue weighting factor [49]. The breast, lung, red bone marrow, stomach, and colon are the most radiosensitive organs, each with a tissue weighting factor of 0.12 (Table 1.1).
Table 1.1
Tissue weighting factors (WT) ICRP 103
Organ |
(WT) |
---|---|
Breast |
0.12 |
Red bone marrow |
0.12 |
Lung |
0.12 |
Colon |
0.12 |
Stomach |
0.12 |
Gonads |
0.08 |
Thyroid |
0.04 |
Esophagus |
0.04 |
Liver |
0.04 |
Bladder |
0.04 |
Bone surface |
0.01 |
Brain |
0.01 |
Salivary glands |
0.01 |
Skin |
0.01 |
Remaindera |
0.12 |
4.1 Biological Effects of Ionizing Radiation
Diagnostic radiation has two biological effects: deterministic (tissue-effect) and stochastic. Deterministic effects have a definite exposure threshold below which there is no effect; once the threshold is exceeded, the severity of the effect correlates to the dose. Classic examples are skin reddening and hair loss, which occur if 2 Gy are delivered to a localized area and which have been reported after excessive radiation during CT brain perfusion studies [50]. Skin reddening and hair loss can also occur after high-dose interventional fluoroscopic procedures [51].
Stochastic effects are random events that have no threshold dose. The probability of their effect increases with higher radiation dose. Radiation-induced cancer data best fits with a linear, no-threshold model, with potential risk of cancer induced by even the smallest dose [52]. It is estimated that for a single exposure of 100 mSv of radiation, one additional cancer per 100 people will develop (as a baseline, 42 people out of 100 will develop cancer) [52].
Ionizing radiation delivered in the course of orthopedic imaging may, therefore, eventually cause malignancy, either as an indirect x-ray interaction of hydroxyl radicals with DNA or as a direct x-ray interaction with DNA. The indirect method is more common, but both interactions can cause a double-stranded DNA break [53]. Children are up to ten times more likely to develop cancer than are middle-age adults (Fig. 1.7) [55]. Furthermore, patients with some inherited diseases have increased sensitivity to radiation (Table 1.2) [56].
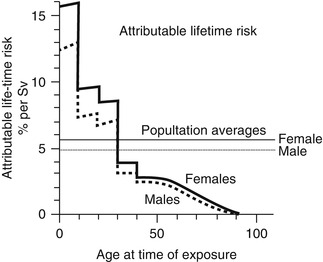
Fig. 1.7
Lifetime risk of excess cancer per sievert as a function of age at the time of exposure (based on data from A-bomb survivors). While the average risk for a population is about 5 % per sievert, the risk varies considerably with age. Children are much more sensitive than adults (Reprinted from Ref. [54], with kind permission from Springer Science+Business Media)
Table 1.2
Selected inherited syndromes associated with increased sensitivity to radiation. This list contains syndromes associated with mutations in DNA repair that increase the risk of developing cancer after ionizing radiation causes DNA damage. There are many other syndromes that are associated with increased risk of cancer including syndromes related to cancer predisposition genes [56]
Ataxia–telangiectasia |
Ataxia–telangiectasia-like disorder |
Seckel syndrome |
Nijmegen breakage syndrome |
Fanconi anemia |
Homologues of RecQ–Bloom syndrome, Werner syndrome, and Rothmund–Thomson syndrome |
Radiation doses over 100 mSv are clearly associated with fatal malignancy, and some argue that doses as low as 10–50 mSv can cause malignancy [57]. The Life Span Study has followed atomic bomb survivors over 60 years; this provides the best stochastic risk data [4, 5]. Studies have also implicated diagnostic imaging. As far back as 1958 [58], in utero exposure was associated with a 6 % increase in absolute risk of cancer per Gy; 10 milligray (mGy) in utero exposure was associated with increased childhood cancer deaths [59]. A study that followed women with scoliosis for 50 years found a 1.69-fold increased incidence of breast cancer deaths (the mean breast dose from scoliosis radiographs was 108 mGy). As the number of radiographs increased, so did the risk of breast cancer death [60]. PA rather than AP frontal views reduce the breast cancer risk by three- to fourfold [61]. Furthermore, recent retrospective pediatric studies have linked CT scans with a small increased incidence of leukemia and brain tumors (one extra case of either per 10,000 head CTs) in one UK study [62] and small increased incidence of multiple types of cancers in an Australian study [63]. More pediatric CT cohort studies examining the radiation risk will be reported in the next few years [64]. These studies are based on actual patient exposure data.
Many high-profile journal articles have used theoretical, population-based risk estimates from low-dose radiation, based on such sources as the Biological Effects of Ionizing Radiation (BEIR) VII report and atomic bomb survivors. For example, Berrington de González and Darby estimated a cumulative cancer risk from diagnostic imaging at 0.6 % in the UK, from 0.6 % to 1.8 % in 13 other developed countries, and at greater than 3 % in Japan [65]. Brenner and Hall estimate that 1–2 % of all future cancers will be caused by CT [66].
Physicists have developed position statements in response to theoretical studies on population-based risk estimates from low-dose radiation. For example, the American Association of Physicists in Medicine (AAPM) cautions that “Predictions of hypothetical cancer incidence and deaths in patient populations exposed to such low doses [less than 100 mSv] are highly speculative and should be discouraged. These predictions are harmful because they lead to sensationalistic articles in the public media that cause some patients and parents to refuse medical imaging procedures, placing them at substantial risk by not receiving the clinical benefits of the prescribed procedures” [67]. Similarly, the Health Physics Society, in “accordance with current knowledge of radiation health risks… recommends against quantitative estimation of health risks below an individual dose of 5 rem [50 mSv] in one year or a lifetime dose of 10 rem [100 mSv] above that received from natural sources” [68].
The International Atomic Energy Agency is attempting to change the focus from collective dose to the population to individual dose protection by launching a Smart Card/SmartRadTrack project that tracks patient examinations and cumulative dose [69]. If technique is known, organ dose and effective dose can be estimated, though precise estimation of the risk from a specific study requires calculations performed by a qualified medical physicist. A simpler approach is comparing the relative risk of radiographs, CTs, fluoroscopy, and NM in order to put the radiation risk from common orthopedic imaging tests in context. The American College of Radiology (ACR) has a relative radiation level scale that provides reasonable estimates of dose (Table 1.3) [70].
Table 1.3
Relative radiation exposure from different imaging studies. Actual patient doses in these procedures vary according to body habitus, the region exposed to ionizing radiation, the number of images, and technique factors. The range of doses is based on the ACR Appropriateness Criteria® Radiation Dose Assessment Introduction [70] and the corresponding ACR Appropriateness Criteria as referenced except for the dose range specified for scoliosis series [71] and PET/CT [72, 73]
Relative radiation level [70] |
Adult effective dose estimate range (mSv) |
Pediatric effective dose estimate range (mSv) |
Example examinations |
---|---|---|---|
O |
0 |
0 |
Ultrasound; MRI |
|
<0.1 |
<0.03 |
Chest radiographs; hand radiographs |
|
0.1–1 |
0.03–0.3 |
Pelvis radiographs, cervical spine trauma series [74] |
|
0.1–1 |
0.03–0.3 |
|
|
1–10 |
0.3–3 |
Scoliosis series (2 views) [71] |
|
1–10 |
0.3–3 |
Tc-99m bone scan |
|
1–10 |
0.3–3 |
Abdomen CT |
|
10–30 |
3–10 |
Whole body PET |
|
30–100 |
10–30 |
Although we do not have precise risk estimates for low-dose radiation, it is prudent to treat ionizing radiation as increasing the risk of developing cancer. If there is no benefit to a study, it should not be performed. If there is a benefit to performing the study, the risks should be mitigated. The “as low as reasonably achievable” (ALARA) principle aims to minimize the risk of cancer and was initially developed to protect medical and dental personnel. ALARA has been adapted for patients. It states that the small risk of developing cancer must be weighed against the benefits of performing an examination that employs ionizing radiation and, when feasible, MRI or US should be performed. The Image Gently® campaign (Alliance for Radiation Safety in Pediatric Imaging, Cincinnati, Ohio) recommends adjusting dose for patient size.
4.2 Radiography
Radiography accounts for the greatest number of all ionizing radiation imaging examinations in children (84.7 %); CT, fluoroscopy, and NM—in descending order of frequency—account for the remainder [75]. Digital radiography has replaced screen-film radiography in many imaging centers. There are two types of digital radiography: computed radiography (CR) and direct digital radiography (DR).
CR uses a photostimulable storage-phosphor imaging plate to create a latent image on a cassette. The cassette is then placed in a laser reader, and a digital image is produced in 30–40 s. Powder phosphor imaging plates are the most common type of CR. More recently, needle phosphors have been developed [76], reducing patient exposure [77].
DR eliminates the need for a separate scanning step because the x-ray detector is bonded directly onto integrated thin-film transistors, allowing the readout to appear in less than 10 s. DR can use either direct detection that converts x-rays into electronic charge or indirect detection that converts x-rays first into light and then into electronic charge.
Digital radiography has many advantages over screen-film radiography. Image storage and distribution permit simultaneous viewing in the radiology suite and in clinical areas. Digital radiography has a latitude of exposure approximately 100 times greater than screen-film radiography [78], reducing the number of repeat examinations due to under- or overexposure. Image manipulation (processing) can enhance subtle characteristics in an image. Although digital images have lower spatial resolution than screen-film radiographs, their improved contrast and image processing can result in superior studies [76].
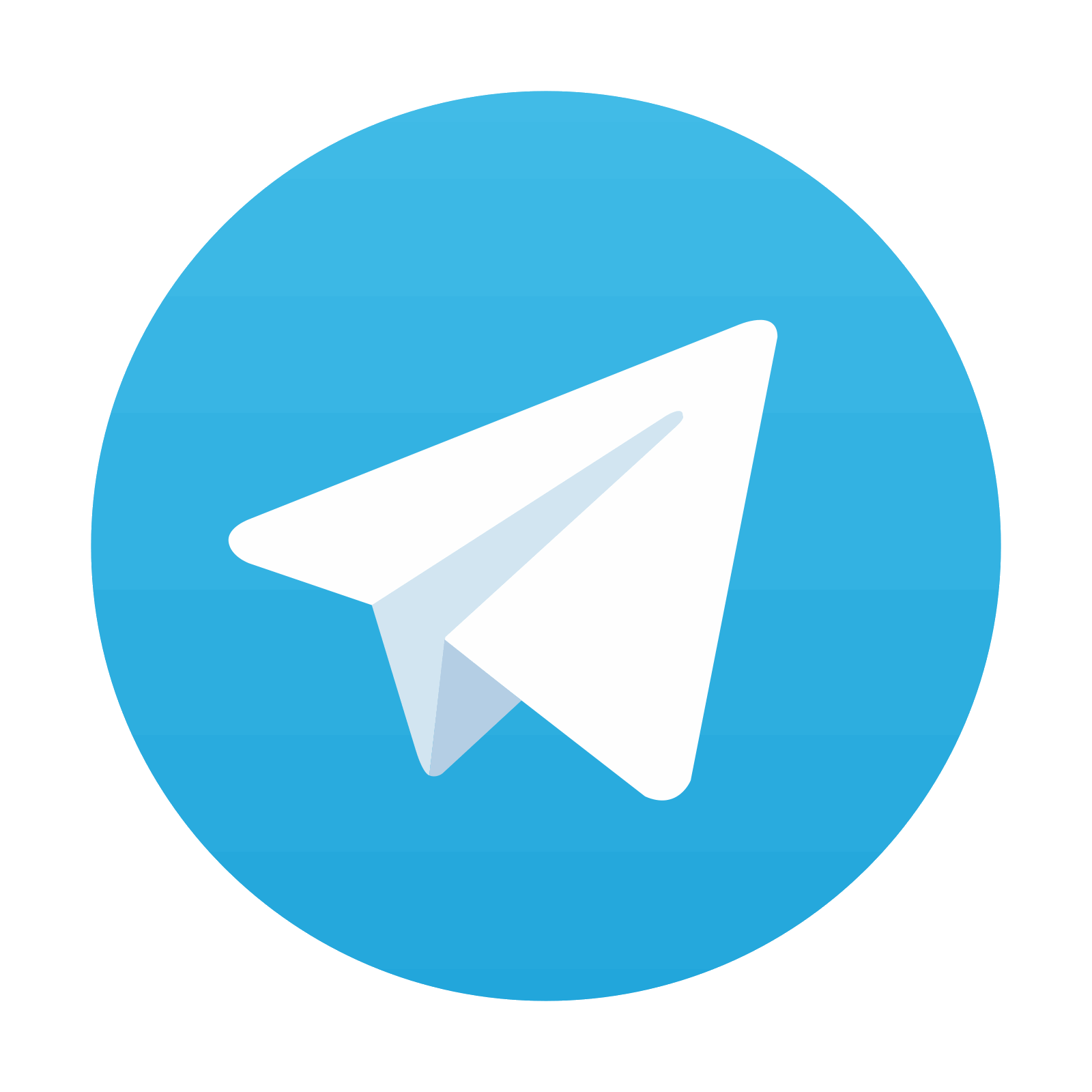
Stay updated, free articles. Join our Telegram channel
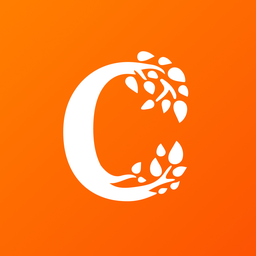
Full access? Get Clinical Tree
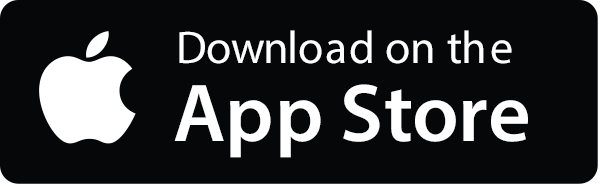
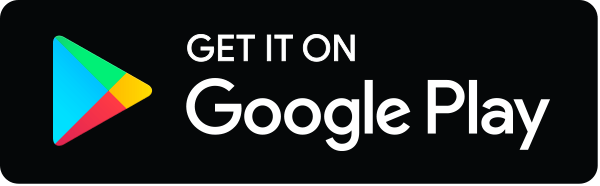