Abstract
In this chapter on myocardial ischemia, the pathophysiology of insufficient oxygen supply is illustrated by different invasive and non-invasive imaging techniques. Anatomical versus functional methods are discussed. Strengths and weaknesses of the most current cardiac imaging methods are listed, but more importantly a diagnostic platform is highlighted; to choose the optimal imaging modality, not only is the context of the patient with his/her complaints, habitus and comorbities important, but local expertise and availability of modalities are important as well. Furthermore, the primary goal of our diagnostic arsenal should be focused on detecting clinically and prognostically relevant ischemia, and on guiding treatment options.
Keywords
Stable ischemic heart disease, Patho-physiology, Coronary and fractional flow reserve, Pretest probability, Diagnostic platform.
Abbreviations
BOLD
blood–oxygen-level-dependent
CAD
coronary artery disease
CFR
coronary flow reserve
CTCA
computed tomography coronary angiography
ECHO
echocardiography
FFR
fractional flow reserve
ICA
invasive coronary angiography
IHD
ischemic heart disease
IVUS
intravascular ultrasound
MR
magnetic resonance
MRA
magnetic resonance imaging angiography
MRS
magnetic resonance spectroscopy
OCT
optical coherence tomography
PET
positron emission tomography
PTP
pretest probability
SCAD
stable coronary artery disease
SPECT
single-photon emission computer tomography
9.1
Pathophysiology of ischemia
Ischemia is caused by the imbalance between oxygen demand by and oxygen supply to the myocardial cells. Oxygen demand is determined by contractility, heart rate, and wall tension (preload and afterload), the latter depending mainly on cavity pressure and size. Oxygen supply occurs exclusively over the coronary circulation: in contrast to many other tissues in the human body, oxygen extraction (arterio—venous oxygen difference) by the cardiomyocytes is near maximal, so that variation in blood supply is the only variable to adjust oxygen delivery and keep it in balance with oxygen demand. The other variable, i.e., the oxygen carrying capacity of the blood, can be abnormal in some diseases (= hypoxemia f.i. due to lung disease or anemia), but in general it is coronary flow and its distribution over the coronary tree that defines oxygen delivery to the myocardial cells. The primary symptom of ischemia is angina, but patients can have ischemia without angina (silent ischemia) and present with other consequences of ischemia, such as arrhythmia, sudden cardiac death, or left ventricular dysfunction. A local release of vasoactive substances, such as adenosine, is induced by ischemia and is thought to play a role in the formation of collaterals and in ischemic preconditioning (cf. Figure 9.1 ).
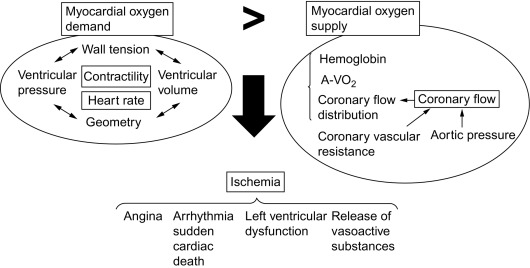
Coronary blood flow is determined by the aortic pressure on one hand, mainly during diastole when intramyocardial pressure is low, and on the other hand, coronary vascular resistance over the entire coronary vasculature, which consists of the coronary epicardial branches (1), the microvasculature (mainly the arterioles) (2) and the capillary bed (3) (cf. Figure 9.2 ). The coronary vascular resistance can be pathologically increased structurally or functionally at each of the three levels, thereby potentially causing ischemia.
- (1)
At the epicardial level, a coronary artery stenosis can be caused by an atheroma, thrombus, and/or vasospasm ( Figure 9.3 ). Atheromatosis is the most prevalent cause of increased coronary vascular resistance impeding oxygen supply. Histologically, a stable atheroma shows less frequent erosion or rupture of the endothelial lining than a vulnerable plaque causing an acute coronary syndrome. The lesions are typically fibrotic, poorly cellular, with small necrotic cores, thick fibrous caps, and little or no overlying thrombus ( Figure 9.4 ) [ ].
Figure 9.3
Mechanisms of myocardial ischemia. In addition to atherosclerotic and vasospastic disease, coronary microvascular dysfunction has emerged as a “third” mechanism of myocardial ischemia. It can occur alone or in combination with atherosclerotic and/or vasospastic disease and can lead to transient myocardial ischemia as in patients with CAD or cardiomyopathy or to severe acute ischemia as observed in Takotsubo syndrome.
From Ref. [ ].
Figure 9.4
Coronary angiography of a 59-year-old man with a stenosis on the mid-LAD (white arrow). OCT shows an intact endothelium over a stable atheroma. FFR was 0.85. The patient was treated conservatively.
With courtesy of Tom Adriaenssens, MD, PhD, University Hospital Leuven, Belgium.
- (2)
In normal coronary vasculature under resting conditions, the microvascular resistance is quite high due to a certain degree of vasoconstriction of the arterioles and the sphincters. This allows for autoregulation of the myocardial blood flow by vasodilation of this microvascular bed to compensate for f.i. an epicardial stenosis or low blood pressure (cf. Figure 9.5 ). Vasodilation can be induced by local release of endothelium-dependent or -independent vasoactive substances, such as respectively nitric oxide or adenosine, resulting in a direct local relaxation of smooth muscle cells in the vessel wall (cf. Figure 9.6 ). Normal endothelial function contributes to vasodilation by nitric oxide release from the endothelium as a response to increased shear stress, whereas endothelial dysfunction will result in vasospasm. A disturbance of the local adenosine metabolism can also be a dysfunctional factor by impaired vasodilation. Increased vasoconstriction, impaired, or inhomogenous vasodilation (steal-effect) in the microvascular bed occurs in f.i. syndrome X patients or in diabetics with microvascular dysfunction [ ].
Figure 9.5
Microvascular resistance. In normal coronary vasculature, the microvascular resistance is quite high by maintaining a certain degree of vasoconstriction of the arterioles and the sphincters. This allows for autoregulation of the myocardial blood flow, by vasodilation of this microvascular bed to compensate for f.i. an epicardial stenosis or low blood pressure.
From Boron & Boulpaep textbook Physiology.
Figure 9.6
Vasorelaxation. Vasodilation can be induced by local release of endothelium-dependent (shear stress) or -independent vasoactive substances, such as respectively nitric oxide or adenosin, resulting in a direct local relaxation of smooth muscle cells in the vessel wall.
- (3)
A relative deficit of capillaries can occur in left ventricular hypertrophy; microvascular obstruction can result from cellular necrosis, edema, inflammation, and/or thrombus as in acute myocardial infarction. Myocardial blood flow depends on coronary blood flow, but local mechanical factors such as myocardial contraction and left ventricular pressure also influence perfusion pressure, resulting in a transmural myocardial perfusion gradient, with the subendocardium being most vulnerable to ischemia.
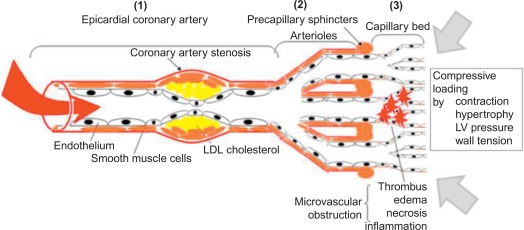
Clinical circumstances causing an increase in oxygen demand are f.i. exercise, stress, emotion, and cold exposure. In case diastolic aortic pressure is low, such as in aortic insufficiency or in elderly with isolated systolic hypertension and left ventricular hypertrophy, ischemia can occur in the absence of a severe epicardial coronary artery stenosis. Also shortening of the duration of diastole at higher heart rate, f.i. in case of anemia or in patients with atrial fibrillation with rapid ventricular response, can cause subendocardial ischemia, especially in hypertrophic ventricles. So the actual occurrence of angina can vary in a given patient and can occur even at rest in patients with stable epicardial lesions or microvascular dysfunction, making it difficult to distinguish these from vasospastic angina or even an acute coronary syndrome, only on the basis of symptoms and the circumstances of their occurrence. Epicardial atherosclerosis and microvascular dysfunction are relatively stable structural and functional alterations, that are associated with fairly stable angina or other ischemic alterations such as ECG-changes, starting at a certain level of exercise intensity.
Vasospasm on the other hand, is a transitory phenomenon that often occurs at rest with preserved effort tolerance (Prinzmetal angina). Vasospasm can occur in apparently normal or atherosclerotic coronary arteries, it can be (multi)focal or diffuse, and it is caused most often by vasoconstrictor stimuli acting on hyperreactive smooth muscle cells, although endothelial dysfunction can reinforce the problem. Invasive administration of acetylcholine provoking vasoconstriction by direct stimulation of smooth muscle cells, instead of vasodilation by NO-release from the endothelium, would indicate endothelial dysfunction, whereas ergonovine administration can induce vasospasm by hyperreactive smooth muscle cell contraction [ ].
Very often these different coronary abnormalities co-exist in one patient and cause ischemia by a combination of effects. A new concept of ischemic heart disease (IHD) puts myocardial ischemia at the center of our attention and critical coronary stenosis, vasospasm, and microvascular dysfunction in the margin as one of many contributors to myocardial ischemia (cf. Figures 9.3 and 9.7 ).
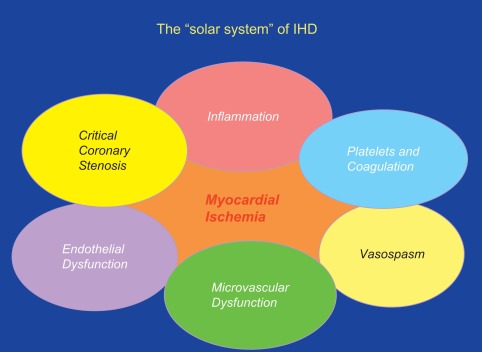
9.1.1
The ischemic cascade
The consequences of a transient imbalance between blood/oxygen supply and metabolic demand occur in a predictable temporal sequence, involving (1) insufficient myocardial perfusion resulting in (2) abnormal metabolism with increased H + and K + concentration in the venous blood that drains the ischemic territory, (3) signs of ventricular diastolic and subsequently systolic dysfunction with regional wall motion abnormalities, (4) development of ST–T changes, and (5) cardiac ischemic pain (angina). This ischemic cascade explains why imaging techniques that identify abnormalities in perfusion, metabolism, or cardiac mechanics are more sensitive than ECG signs or symptoms in detecting ischemia (cf. Figure 9.8 ).
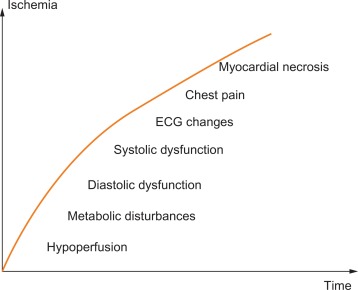
9.2
Invasive diagnosis of ischemia
9.2.1
Anatomy of coronary artery disease
Invasive coronary angiography (ICA) remains the “gold standard” in depicting epicardial coronary artery disease (CAD). However, the imaging information is only about the lumen, and not the plaque, let alone the consequences of the structural abnormality.
This plaque can be characterized invasively by intravascular ultrasound (IVUS) or optical coherence tomography (OCT). IVUS demonstrates the full thickness of the plaque (except in the presence of extensive subintimal calcification), but its resolution is insufficient to measure cap thickness, whereas OCT penetration is much more limited (1 mm), but its greater resolution allows for reliable identification of subintimal lipidic plaques and precise measurement of the fibrous cap, the two key elements characterizing vulnerable plaques (cf. Figure 9.6 ). Both techniques have greatly added to our understanding of the natural history of coronary atherosclerosis and offer, to some extent, “virtual histology” (cf. Chapter 8 ).
Alternatively, coronary anatomy may be visualized noninvasively by coronary computed tomography angiography (CTCA) or magnetic resonance imaging angiography (MRA) (see Chapter 5 , Chapter 6 , Chapter 7 , Chapter 8 ).
All these anatomical techniques provide information about the lumen and/or the plaque surrounding the lumen but do not address function of the epicardial coronary arteries or the condition of the microvasculature.
9.2.2
Ischemia
In stable patients, diagnosing the presence of CAD should be differentiated from diagnosing the presence of ischemia, caused by obstructive CAD and/or microvascular dysfunction [ ]. Due to the lack of a linear relationship between epicardial coronary artery stenosis and coronary flow, there is a need for a functional evaluation of the severity of epicardial stenoses and microvascular dysfunction. The more variable causes of ischemia, i.e., vasospasm, warrant a specific diagnostic scheme using provocation tests.
Two methods to invasively determine the functional significance of a lesion are coronary flow reserve (CFR) and fractional flow reserve (FFR). Although these concepts are related, they measure different components of the system of epicardial vessels, microvasculature, and the dependent myocardium. More recently, an instantaneous wave-free ratio (iFR) has shown to be equivalent to FFR, without the need for adenosine administration [ ].
The absolute CFR is defined as the ratio of hyperemic to basal coronary blood flow and can be measured invasively by Doppler velocity or thermodilution methods, or noninvasively by measuring Doppler velocities echocardiographically or by measuring myocardial perfusion reserve (ratio of stress over rest perfusion) quantitatively or semiquantitatively with positron emission tomography (PET), magnetic resonance imaging, or transthoracic contrast echocardiography (ECHO).
In a patient with an epicardial coronary artery stenosis, the release of ischemic metabolites, such as adenosine, within the under-perfused myocardium downstream to the stenotic artery, results in dilation of the distal arterioles and precapillary sphincters. This compensatory vasodilation favors local perfusion but at the price of “consuming” part of the normally available flow reserve. At a perfusion pressure of about 50 mmHg, the coronary resting flow is preserved due to maximal downstream vasodilatation but no further flow reserve is available (cf. Figure 9.9 ).
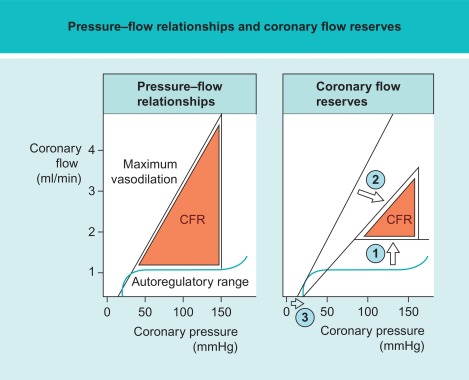
Due to numerous influencing factors, CFR has a high interindividual variability (cf. Figure 9.10 ). Healthy subjects have an absolute CFR of 3.5–5, whereas patients with a significant epicardial stenosis have a CFR ≈ 2–2.5. Patients with a CFR < 2 have an adverse prognosis, even in the absence of epicardial disease, because in that case CFR indicates severe microvascular disease [ , ]. Flow reserve values between 2.5 and 3.5 are difficult to interpret but may indicate milder forms of coronary microvascular dysfunction, with and without associated epicardial disease. In this respect it is important to take into account the presence of diffuse disease and coronary artery remodeling. Diffuse disease both in the absence or presence of any focal stenosis will have a significant supplementary negative impact on CFR. Adaptive remodeling on the other hand, will partially “compensate” for the presence of a focal stenosis and reduce the impact on CFR [ ].
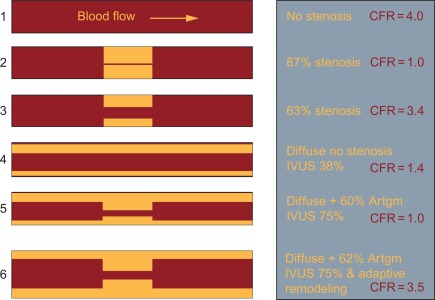
The complexity of interpreting this “multi-factorial” CFR led to the use of the more simple FFR method in determining the significance of an epicardial stenosis, making to a large extent abstraction from distal microvasculature. The FFR is defined as the ratio of the maximal hyperemic flow in the diseased epicardial coronary artery over the hypothetical maximal hyperemic flow in this artery in case there was no disease. It is based on the assumption that at maximal vasodilation, vascular resistance of the coronary artery is minimal and constant for both the hypothetical and the diseased situation, so that coronary flow is mainly driven by pressure. In a normal coronary artery, this pressure equals aortic pressure, whereas in a diseased coronary artery, the pressure drops over the epicardial stenosis and therefore equals the pressure measured distally of this stenosis. FFR is then defined as the ratio of the post-stenotic over the aortic pressure at hyperemia, which can be measured invasively and can easily be interpreted. When the FFR becomes ≤ 0.8, downstream perfusion may be inadequate. Again, there is no linear relationship between the severity of an angiographic stenosis and the reduction in FFR it causes, stressing the inadequacy of mere anatomical evaluation to identify the impact on perfusion (cf. Figure 9.4 ).
9.2.3
Relationship between myocardial perfusion and FFR
How, then, is the relationship between CFR (which represents an integrated parameter of myocardial perfusion) and FFR? As can be seen in Figure 9.11a , no clear CFR–FFR relationship is found in this broad scatter. This indicates that, although FFR is a functional measurement of ischemia as compared to the anatomical methods, it does not reflect microvasculature and distal coronary flow. Therefore, noninvasive imaging modalities measuring myocardial perfusion can still differ from FFR as a “gold standard” for the detection of ischemia, due to fundamental differences in pathophysiology, rather than shortcomings of methodology [ ]. However, by taking into account the presence of diffuse disease, the CFR–FFR relationship is revealed as illustrated in the next graph ( Figure 9.11b ). From this pathophysiologic framework, it is clear that in case FFR is < 0.8 and CFR is < 2, both values are concordantly reduced and the patient would benefit from revascularization, whereas a FFR > 0.8 and CFR > 2 would indicate a nearly normal situation, making revascularization unnecessary. In case there is a FFR > 0.8, but CFR < 2, angiography mostly confirms diffuse coronary disease rather than a focal stenosis, making this patient not eligible for a revascularization procedure. This leaves us with the situation of a lesion with FFR < 0.8 but CFR > 2, indicating a significant focal lesion, but adequate CFR, possibly due to positive, adaptive remodeling, where one could question the necessity to intervene on the lesion.
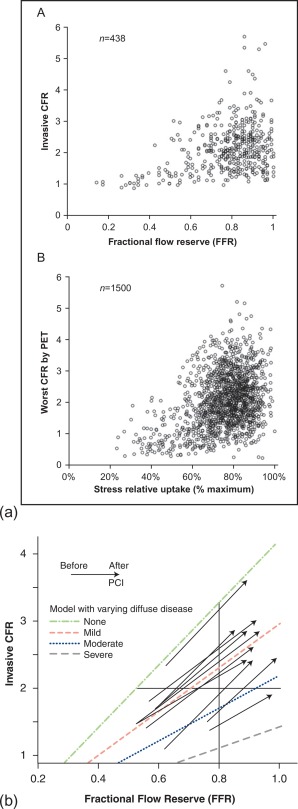
FFR-guided PCI has been shown to be superior to angiographic-guided PCI (based on angiographic judgment of stenosis severity alone) in patients with multivessel disease, for the endpoint of death, nonfatal myocardial infarction, and repeat revascularization at 1 and 2 years (FAME 1) [ , ]. In coronary artery stenoses with FFR < 0.8, revascularization by PCI on top of optimal medical therapy (OMT) was shown to be superior to OMT alone at 1 and 2 years of follow-up, by decreasing the need for urgent revascularization (FAME 2) [ , ]. Recently, the use of FFR has been upgraded to a Class IA recommendation in multivessel PCI in the ESC Guidelines on coronary revascularization [ ].
The decision whether to revascularize a patient should be based on the presence of a significant obstructive coronary artery stenosis, the amount of related ischemia, and the expected benefit to prognosis and/or symptoms. There are many clinical, anatomical, technical, and environmental factors that contribute to this decision-making. Therefore, the treatment should preferentially be an individualized clinical judgment with consensual decision of a heart team [ ].
9.2.4
Heart team discussion
A heart team consists of an interventional cardiologist, a cardiac surgeon, and, according to the European guidelines, preferentially also a noninvasive cardiologist to obtain integrated, active decision making between groups of physicians with diverse expertise and the patient. In certain instances, other providers such as primary care physicians, intensivists, or anesthesiologists can also be involved.
Although no studies have proven superiority of using a heart team instead of making decisions by ourselves, guidelines recommend heart team discussions because people working together in a multidisciplinary manner may reach better decisions by consensus than any individual alone, especially when it involves weighing complicated treatments, such as coronary revascularization, with various risks and benefits. Furthermore, using a heart team can improve timeliness and consistency of decisions when multiple providers are likely to be involved, allow better coordinated treatment plans to be developed (e.g., “hybrid” revascularization procedures), minimize concerns related to physician self-referral, enhance patient enrollment in research protocols, and increase educational opportunities.
9.3
Noninvasive detection and quantification of ischemia
9.3.1
The concept of pretest probability
Recommendations to decide on the appropriateness of the various diagnostic modalities as well as to establish the prognosis of the patient and make the appropriate therapeutic choices rely heavily on estimates of the prevalence of significant CAD in populations characterized by sex, age, and symptoms. On the other hand, the patient with his/her comorbidities and quality of life contributes to the likelihood of potential revascularization.
When there is no indication for a specific ischemia treatment (primary prevention), risk charts such as SCORE or Framingham can be applied to decide on the need for risk modification [ ]. When CAD is suspected and a revascularization treatment is considered if appropriate, an estimation of CAD-probability is relevant for further diagnostic and/or treatment strategy planning. The pretest probability (PTP) conforms to a Bayesian approach, allowing for the calculation of an individualized post-test probability. By using a diagnostic test (f.i. stress imaging modality), an intermediate and therefore indecisive PTP is turned into a low or high post-test probability guiding further treatment. Recent estimates based on CTCA registries of the prevalence of obstructive epicardial CAD in patients with typical or atypical angina are substantially lower than the Diamond and Forrester estimates from 1979, though (cf. Figure 9.12 ) [ ]. In contrast, in patients with non-anginal chest pain, the prevalence of obstructive CAD as assessed by coronary CTCA may be higher than previously expected. In fact, these coronary CTCA data suggest that there may be little difference in the prevalence of obstructive CAD across the three groups of chest pain. So using PTPs from registries with referred patients may overestimate the true PTP in patients presenting in a primary care environment.
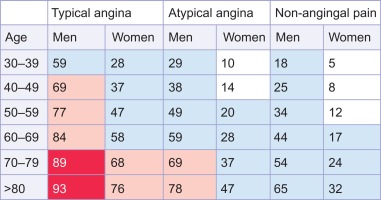
If the pretest probability (PTP) is less than 15% , other causes for symptoms should be explored, including functional coronary disease, and the risk profile of the patient should be established, as mentioned before, to decide on primary preventive measures.
If the PTP is more than 85% the diagnosis of stable CAD is so likely that one can immediately proceed to risk stratification to decide on therapy (revascularization or not).
The 15% and 85% cut-offs follow from the overall sensitivity and specificity of imaging-based diagnostic methods lying around 85%. This means that 15% of all tests will be false, so that it is better in patients with a PTP below 15% or above 85% NOT to perform a test.
In the intermediate PTP between 15% and 85% , noninvasive diagnostic testing is appropriate to convert intermediate PTP into low or high post-test probability (respectively low and high likelihood of CAD), guiding further treatment (cf. Figure 9.13 ) [ ].
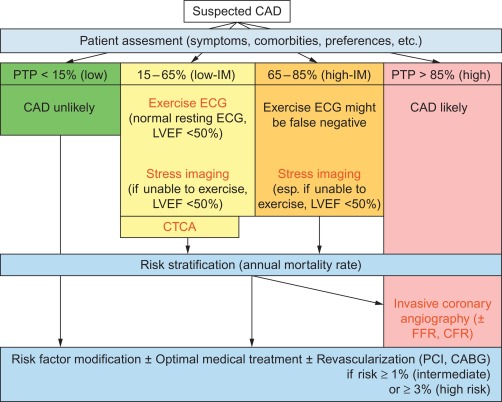
Multiple factors contribute to the decision of which diagnostic test will be best in answering the diagnostic question on the one hand, in the particular patient with his/her comorbidities on the other hand. As a general guide in choosing the stressor, exercise is preferred in case a patient is able to exercise, except if there are already significant wall motion abnormalities at rest (dobutamine preferred) or LBBB (a vasodilator such as dipyridamole preferred).
In case the patient has a low-intermediate PTP between 15% and 50% , CTCA can be used to rule out significant disease. This can be of particular interest in women, where exercise ECG has less diagnostic accuracy compared with men, which is in part related to impaired exercise capacity, but also to ST-segment abnormalities due to hormonal changes, and lower QRS voltage. With current CT technologies for reducing radiation dose, CTCA can be routinely acquired using 1 mSv or less of radiation.
In patients able to exercise, with a low-intermediate PTP of 15–65% and a normal resting ECG and LVEF ≥ 50%, exercise ECG is a good option, but even in these patients stress imaging is preferred if available. In case the LVEF is < 50%, exercise stress imaging is preferred and exercise ECG is only considered when stress imaging is not available. In patients able to exercise, with a high-intermediate PTP of 65–85% , exercise stress imaging is preferred. In patients who cannot exercise, pharmacological stress imaging is preferred if PTP is between 15% and 85% (cf. Figure 9.13 ).
If the diagnostic test is negative, turning the intermediate PTP into a low post-test probability of CAD, further strategy is as for patients with a low likelihood of CAD : other causes for symptoms should be explored, including functional coronary disease, and the risk profile of the patient should be established, as mentioned before, to decide on primary preventive measures. Optimal medical treatment can be considered in case functional coronary disease is likely.
If the diagnostic test is positive, the diagnosis of CAD is established and further strategy is as for patients with a high likelihood of CAD from the start: patient factors and risk stratification will determine the opportunity of the patient being revascularized. If the patient does not want any invasive investigation or treatment, risk factor modification and optimal medical treatment will be offered. If the patient’s comorbidities allow for invasive strategy and revascularization, and if the patient agrees to this strategy if appropriate, then ICA can be performed provided that risk stratification is not low (annual mortality rate < 1%; cf. Figure 9.14 ).
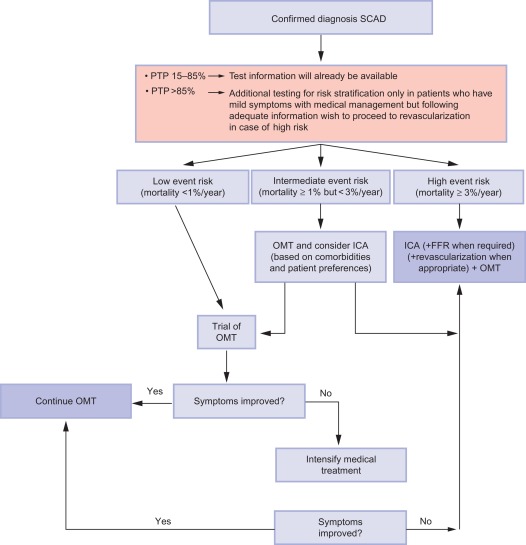
If the diagnostic test is unclear, the choice between a second noninvasive test or ICA is to be made. In case a CTCA was indecisive, f.i. because of technical problems or in patients with heavy calcifications (Agatston score > 400), one should proceed to stress imaging, because the information obtained will also serve risk stratification. In case a stress imaging modality was indecisive, depending on the patient and the circumstances, a second stress imaging modality or a CTCA or ICA can be performed.
9.3.2
Stress ECG
As mentioned before, ischemia gradually occurs in a predictable temporal sequence, with the development of ST–T changes rather late in the ischemic cascade. This explains why ECG signs are less sensitive in detecting ischemia (cf. Figures 9.8 and 9.15 ). Exercise ECG is a completely noninvasive, broadly available, low-cost, well-established and time-honored technique, and despite its inferior performance as compared with modern stress imaging techniques, it is still indicated for a low-intermediate PTP of 15–65% in patients with a normal resting ECG; however, the guidelines do underline the superiority of noninvasive stress imaging when local expertise and availability permits their use (cf. Figure 9.13 ). One must, on the other hand, acknowledge that there are no prospective, randomized data demonstrating that this superior diagnostic performance translates into superior outcomes [ , ].
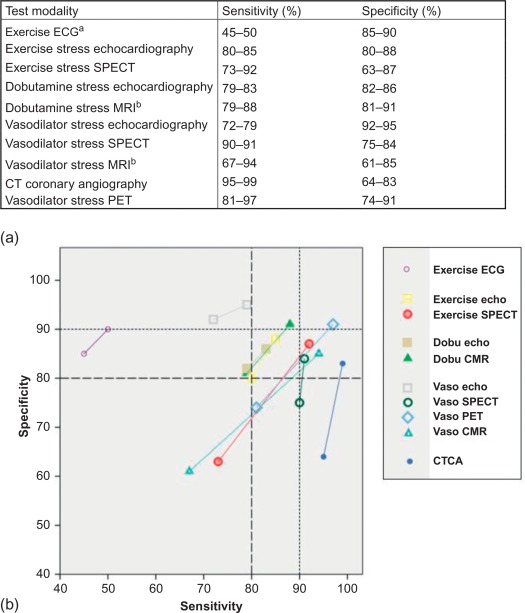
9.3.3
Noninvasive imaging modalities
9.3.3.1
Metabolism
In the ischemic cascade, insufficient myocardial perfusion results in abnormal myocardial metabolism quite early. Therefore, imaging modalities detecting abnormalities in myocardial metabolism are theoretically expected to have high sensitivity for ischemia.
Cardiac magnetic resonance spectroscopy
On average, the heart cycles 10 tons of blood each day using 100,000 heartbeats, resulting in an enormous ATP requirement of about 6 kg a day. Free fatty acids and glucose contribute to ATP synthesis in a ratio of 3:1 in normal situations. The creatine kinase system (PCr) acts as an important energy buffer, providing the heart with energy when the demand outweighs the supply. Such a situation results in a decreased PCr concentration and increased ADP concentration, whereas ATP concentrations are held constant.
MRS is able to measure PCr and ATP-peaks. The PCr peak and a combination of the three peaks of ATP are used to calculate the PCr/ATP ratio. This ratio is the most commonly used parameter in 31P-MRS of the heart. In ischemia, ATP synthesis cannot meet the ATP requirement, resulting in a decrease in the PCr/ATP ratio.
MRS of the heart has been performed using 1.5 and 3.0 T magnetic resonance (MR) systems, and recently even using ultra-high 7 T MR systems.
A major drawback of the application of cardiac magnetic resonance spectroscopy (CMRS) in general, although significant in IHD, is the low spatial resolution. Since IHD does not involve the whole myocardium, information on localization of the ischemic or infarcted area is hard to establish with the current techniques. Overcoming these issues with, for example, higher field imaging or hyperpolarization could pave the way for CMRS to be of diagnostic relevance, most notably in patients with suspected IHD without coronary artery stenosis on ICA. Moreover, CMRS could be of use in patients to discriminate between viable and non-viable myocardium [ ]. Presently, however, CMRS is a research tool with no established clinical indications.
Positron emission tomography, single-photon emission computed tomography
Ischemia may cause reduction of fatty acid utilization and shift from fatty acid to glucose utilization. Such metabolic shift may persist shortly after recovery of ischemia. Glucose metabolism is most easily imaged using 2-fluorodeoxyglucose (FDG) labeled with fluorine-18 ( 18 F). Free fatty acids (e.g., palmitic acid), but also acetate have been labeled with carbon-11 ( 11 C), limiting their use in clinical practice. Acetate has been proposed as a tracer of oxidative metabolism because its early rapid clearance from the myocardium is related to oxygen consumption. PET imaging combining perfusion with myocardial metabolism using 18 F-fluorodeoxyglucose ( 18 F FDG) is an accurate standard for assessment of myocardial hibernation and risk stratification of patients with left ventricular dysfunction of ischemic etiology.
15-( p -Iodophenyl)-3 R , S -methyl pentadecanoic acid (BMIPP) is an iodinated branch-chain fatty acid used by single-photon emission computer tomography (SPECT), showing BMIPP uptake in ischemic myocardium. Ischemia shifts the source of myocardial energy from aerobic metabolism driven by fatty acid oxidation to anaerobic metabolism, where glucose utilization through anaerobic glycolysis becomes pivotal for energy production. These metabolic changes are imprinted onto ischemic myocardium for up to 30 h after the ischemic episode and can be visualized by reduced BMIPP uptake, a phenomenon termed “ischemic memory.” According to a review of mostly retrospective studies, an abnormal finding on BMIPP imaging is significantly associated with future cardiovascular outcomes across the spectrum of CAD, with BMIPP imaging with the patient at rest being particularly useful for the risk stratification of patients with acute chest pain [ ].
Nitrate-enhanced Tc-sestamibi and thallium redistribution imaging are widely used for viability assessment, but metabolic imaging techniques are rarely used to detect myocardial ischemia in clinical practice.
9.3.3.2
Anatomy
Computed tomography coronary angiography-derived ischemic parameters
Computed tomography coronary angiography (CTCA) is by nature an anatomical test with accurate information of the presence and severity of coronary atherosclerotic lesions.
State of the art technology requires at least 64-slice CT [ ]. The technique has high sensitivity and negative predictive value for the detection of angiographic CAD (cf. Figure 9.15 ) [ ]. The assessment of coronary artery bypass grafts is highly accurate, but the evaluation of the native coronary vessels in post-bypass patients is difficult and prone to false positive findings [ , ]. The technique is also less reliable in patients with stents [ ]. CTCA allows for quantification of atherosclerotic plaque burden and obstructive CAD, particularly in the proximal segments. It therefore carries an incremental prognostic value beyond clinical risk factors [ ]. For a more detailed description on the role of CTCA the reader is referred to Chapters 5 and 7 of this textbook.
Functional information on hemodynamic severity of coronary lesions currently escapes standard CTCA, unless adjunctive novel techniques such as CTCA FFR or CT perfusion (CTP) are employed. In CT-FFR software creates a 3D model of the coronary tree from ordinary CTCA examinations with no modification to imaging protocols, no additional image acquisition, and no additional radiation (see Chapter 5 ) [ ]. Maps of coronary blood flow are created computationally, based on principles of myocardial mass and resting coronary flow applied to the measurement of vessel size at CT, in order to demonstrate the flow impact of a stenosis ( Figure 9.16 ). Current CT-FFR applications require transfer of data to an off-site laboratory, several hours of processing on a supercomputer, and a turn-around time of approximately 24 h. CT-FFR was compared against the gold standard invasive FFR in 252 patients in the DeFACTO trial, showing a significant improvement in accuracy, specificity, and positive predictive value, with no sacrifice in sensitivity and negative predictive value compared to CTCA [ ]. The findings suggest that CT-FFR could potentially eliminate the need for risky and costly invasive evaluation and treatment in some patients, especially those at intermediate risk of heart disease, leading to better clinical outcomes and lower costs. Nonetheless, the DeFACTO trial failed to reach the primary efficacy endpoint defined as a diagnostic accuracy significantly above 70% compared to invasive FFR. Recently, a second updated CT-FFR software version with improved image segmentation and refined physiological models has been released and validated in the HeartFlow-NXT trial [ ]. Adding CT-FFR to CTCA alone increased particularly the specificity (from 34% to 79%) and positive predictive value (40–65%) for diagnosing flow-limiting coronary lesions.
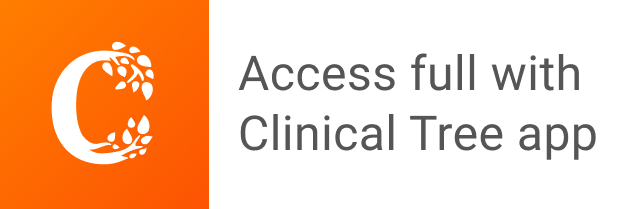