Myocardial Neurotransmitter Imaging
Markus Schwaiger
Ichiro Matsunari
Frank M. Bengel
The innervation of the heart represents an important aspect for the adaptation of cardiac performance to the hemodynamic requirements in the healthy and diseased human body. The cardiovascular work of the normal heart depends on neuronal input for its adequate response to physiologic stimuli such as exercise and mental stress. The importance of the autonomic nervous system (ANS) in the pathophysiology of various heart diseases, such as congestive heart failure (CHF) (1) and arrhythmias (2), has been increasingly recognized. With the introduction of tracer approaches, noninvasive functional assessment of the cardiac ANS by scintigraphic techniques has become a practical reality and provided important pathophysiologic information in various cardiac disease states.
The catecholamine analogue iodine-123 ([123I]) metaiodobenzylguanidine (MIBG) (3,4) has opened noninvasive assessment of presynaptic neuronal function for broader clinical application using single-photon emission computed tomography (SPECT). Tracers for positron emission tomography (PET) are also available to assess the human sympathetic nervous system with carbon-11 ([11C])-hydroxyephedrine (HED) (5,6), fluorine-18 ([18F])-fluorodopamine (7,8), [11C]-epinephrine (EPI) (9), [11C]-phenylephrine (10), and [11C]-CGP12177 (11). These agents permit the regional quantification of tracer concentration in pre- and postsynaptic sites with high spatial resolution.
Description of the Autonomic Nervous System in the Heart
The ANS, referred to as the visceral nervous system, consists of two main divisions: sympathetic and parasympathetic innervation. The two systems have different major neurotransmitters, norepinephrine or acetylcholine (ACh), which define the stimulatory and inhibitory physiologic effects of each system (12). Sympathetic and parasympathetic innervation of the heart facilitates electrophysiologic and hemodynamic adaptation to changing cardiovascular demands. Both sympathetic and parasympathetic activity control the rate of electrophysiologic stimulation and conduction, whereas contractile performance is primarily modulated by sympathetic neurotransmission. This functional characterization is reflected by the anatomic distribution of sympathetic and parasympathetic nerve fibers and nerve terminals.
Sympathetic nerve fibers are characterized by multiple nerve endings that are filled with vesicles containing norepinephrine. Sympathetic nerve fibers travel parallel to the vascular structures on the surface of the heart and penetrate the underlying myocardium in much the same fashion as the coronary vessels. Based on tissue norepinephrine concentration, the mammalian heart is characterized by dense adrenergic innervation with a norepinephrine concentration gradient from the atria to the base of the heart and from the base to the apex of the ventricles (13).
In contrast to sympathetic nerve fibers, parasympathetic innervation is most prevalent in the atria of the heart, the atrioventricular node, and to a lesser degree within the ventricular myocardium. Parasympathetic fibers in the ventricles appear to travel close to the endocardial surface, in contrast to sympathetic innervation.
The enzyme choline acetyltransferase (ChAT) has been used as a reliable marker of cholinergic innervation (14). ChAT concentration is highest in the atria and decreases sharply in both the right and left ventricular myocardium. Fig. 11.4.1 depicts norepinephrine and ACh synthesis in the sympathetic and parasympathetic nerve terminal, respectively.
Sympathetic Nerve Terminal
Norepinephrine, the dominant transmitter in the sympathetic nervous system, is synthesized from the amino acid tyrosine by several enzymatic steps (7). The generation of DOPA from tyrosine is the rate-limiting step in the biosynthesis of catecholamines. After
DOPA conversion to dopamine by DOPA decarboxylase, dopamine is transported into storage vesicles by an energy-requiring mechanism. Norepinephrine is synthesized by the action of dopamine b-hydroxylase (DBH) on dopamine within the storage vesicles. Nerve stimulation leads to norepinephrine release, which occurs as the vesicles fuse with the neuronal membrane and expel their contents by exocytosis. Single nerve stimulation, however, leads to exocytosis of only a small fraction of the many thousands of storage vesicles in the sympathetic nerve terminal.
DOPA conversion to dopamine by DOPA decarboxylase, dopamine is transported into storage vesicles by an energy-requiring mechanism. Norepinephrine is synthesized by the action of dopamine b-hydroxylase (DBH) on dopamine within the storage vesicles. Nerve stimulation leads to norepinephrine release, which occurs as the vesicles fuse with the neuronal membrane and expel their contents by exocytosis. Single nerve stimulation, however, leads to exocytosis of only a small fraction of the many thousands of storage vesicles in the sympathetic nerve terminal.
![]() Figure 11.4.1. Schematic display of sympathetic and parasympathetic nerve terminals. NE, norepinephrine; TYR, tyrosine; MAO, monoamine oxydase; ACh, acetylcholine; AChE, acetylcholinesterase. |
The average adrenergic neuron has approximately 25,000 vesicles. Each vesicle contains approximately 250 pg of norepinephrine (15). Thus, although most norepinephrine is thought to be released by exocytosis, nonvesicular release also occurs. Apart from neuronal stimulation, norepinephrine release is also regulated by a number of receptor systems. The α2-receptors on the membrane surface are thought to provide negative feedback of the exocytotic process, and thus, the exocytotic release can be inhibited by presynaptic a2-receptor agonists such as clonidine, guanabenz, and guanfacine (16).
Muscarinic and adenosine receptors have an antiadrenergic effect in the heart. Neuropeptide Y is stored and released together with norepinephrine from the nerve terminal. Neuropeptide Y is thought to inhibit norepinephrine release by the nerve terminal (17). Presynaptic angiotensin II receptors and b-receptors, on the other hand, mediate facilitation of norepinephrine release from sympathetic nerve endings. Therefore, some antihypertensive pharmaceuticals such as angiotensin-converting enzyme inhibitors and nonselective b-blocking agents can inhibit excessive norepinephrine release.
The complex modulation of sympathetic neurotransmission obviously involves many systems including dopamine, prostaglandin, and histamine. Only a small amount of the norepinephrine released by the nerve terminal is actually available to activate receptors on the myocyte surface. Most of the norepinephrine released undergoes reuptake in the nerve terminal (uptake-1 mechanism) and recycles into the vesicles or is metabolized in the cytosol of the nerve terminal. The uptake-1 system is characterized as saturable and sodium, temperature, and energy dependent (18). It can be inhibited by cocaine and desipramine (19). Structurally related amines such as EPI, guanethidine, and metaraminol are also transported by this system.
In addition to neuronal uptake via uptake-1, the uptake-2 system moves norepinephrine into nonneuronal tissue (20). Uptake-2 is characterized as nonsaturable and not sodium-, temperature-, or energy-dependent. It can be inhibited by steroids and clonidine (21). Free cytosolic norepinephrine is degraded rapidly to dihydroxyphenylglycol by monoamine oxidase (MAO). Only a small fraction of the released norepinephrine diffuses into the vascular space where it can be measured as norepinephrine spill over in the coronary sinus venous blood.
Parasympathetic Nerve Terminal
ACh is synthesized within the parasympathetic nerve terminal. Choline is transported into the cytosol of the nerve ending via a high-affinity choline uptake system (22). In the nerve terminal, choline is rapidly acetylated by ChAT and is subsequently shuttled into storage vesicles. In contrast to amine uptake in the sympathetic nerve terminals, the choline uptake system is restrictive. Even choline analogues with very similar structures are poor substrates for this uptake mechanism. Upon nerve stimulation, ACh is released into the synaptic cleft where it interacts with muscarinic receptors. Free ACh is rapidly metabolized by acetylcholinesterase.
Radiopharmaceuticals for Neurotransmitter Imaging
Presynaptic Function
Tracers for neurotransmitter imaging have been developed by either direct labeling of the physiologic neurotransmitter or labeling of their structural analogues (Fig. 11.4.2). Table 11.4.1 summarizes currently available radiopharmaceuticals for the scintigraphic evaluation of the presynaptic nervous function. Iodine-123 MIBG was developed as a norepinephrine analogue by Wieland et al. (4); it was first used in the human heart by Kline et al. (3) in the early 1980s. This compound is an analogue of guanethidine and shares a cellular uptake mechanism similar to norepinephrine at the sympathetic nerve terminals. It is transported into cells by uptake-1 and is stored in the vesicles but not catabolized by MAO or catechol-O-methyltransferase (COMT). This compound has a low affinity for postsynaptic adrenergic receptors and thus has little pharmacologic action. It has been shown that tissue norepinephrine concentration correlates with MIBG uptake (23).
Because PET has several advantages over SPECT (e.g., quantification of regional tracer uptake with the use of accurate attenuation correction and higher spatial resolution), efforts have been made to develop positron-emitting tracers for neurotransmitter imaging.
Fluorine-18-labeled metaraminol, which also was first synthesized by Wieland et al. (24) at the University of Michigan, is taken up by the sympathetic nerve terminal in a manner similar to norepinephrine but is not catabolized by MAO. Imaging studies in regionally denervated canine hearts indicated sufficient suitability of the tracer to quantitatively assess the integrity of myocardial sympathetic innervation. Transient myocardial ischemia, again in the canine model, resulted in reduced [18F]-metaraminol retention in tissue, which is consistent with neuronal dysfunction in reversibly damaged myocardium (25). Radiopharmaceutical problems associated with low specific activity and potential pharmacologic effects, however, limited its further clinical application.
Carbon-11-HED, also introduced by the Michigan group, emerged as a more promising tracer because it can be synthesized with high specific activity (26). Experimental studies have indicated that there is a highly specific uptake into sympathetic nerve terminals with little nonneuronal binding (27). Carbon-11-HED is currently the most successfully used positron-emitting tracer for cardiac neurotransmitter imaging in humans. More recently, [11C]-EPI has been proposed as a truly physiologic tracer (28). It has been demonstrated that, as with norepinephrine, accumulation of [11C]-EPI by the heart reflects mainly vesicular storage in the sympathetic neuron (29). In contrast to [11C]-HED, free [11C]-EPI is metabolized in the cytosol by the MAO system. Because [11C]-HED is not metabolized, it diffuses out of the nerve terminal and undergoes reuptake.
Myocardial retention of [11C]-HED primarily reflects uptake-1 activity and to a lesser degree the storage capacity of neurons for
norepinephrine (27,30). Therefore, [11C]-EPI may be the more suitable tracer for the evaluation of sympathetic vesicular function of the heart (29). Because [11C]-EPI is metabolized by MAO and COMT, careful consideration should be given to the influence of metabolic pathways on measurements of [11C] retention attributed to vesicular storage functions.
norepinephrine (27,30). Therefore, [11C]-EPI may be the more suitable tracer for the evaluation of sympathetic vesicular function of the heart (29). Because [11C]-EPI is metabolized by MAO and COMT, careful consideration should be given to the influence of metabolic pathways on measurements of [11C] retention attributed to vesicular storage functions.
Table 11.4.1 Radiopharmaceuticals Used for Neurotransmitter Imaging | |||||||||
---|---|---|---|---|---|---|---|---|---|
|
A further analogue of EPI is [11C]-phenylephrine, which also has been synthesized by Del Rosario et al. (31) at the University of Michigan. This tracer enters the nerve terminal via uptake-1, is stored within vesicles, but is also metabolized by the MAO enzyme system. Carbon-11-phenylephrine, therefore, allows for the evaluation of vesicular and enzymatic integrity of the nerve terminal (10,32). Other potential tracers include [18F]-fluorodopamine (7,33) and [18F]-fluoronorepinephrine (34). Although the available clinical and experimental data for these tracers are still limited, the longer physical half-life of [18F] may allow for washout analysis to assess sympathetic nerve tone. Further PET tracers for assessing sympathetic nerve function, such as bromine-76 ([76Br])-labeled or [18F]-labeled benzyl guanidine (35,36), analogues of MIBG, have also been proposed but did not reach a clinical application.
To date, only a few studies have addressed myocardial parasympathetic neuronal imaging, probably because of a low specificity for neuronal uptake and storage. However, recent studies in the brain in mice (37) and rats (38) have shown that iodine-125 ([125I])-labeled iodobenzovesamicol allowed scintigraphic assessment of cholinergic nerve terminals. Fluorine-18-fluoroethoxybenzovesamicol was developed for parasympathetic neurotransmitter imaging using PET (39). The myocardial retention of the tracer was low because of the low cholinergic neuron density, limiting its potential as an imaging agent. Further efforts in developing tracers for the parasympathetic nervous system are required.
Postsynaptic Receptor Imaging
The sympathetic neurotransmission in the heart primarily involves adrenoceptors of type b1 and b2, which are located on myocardial cells. The a-adrenoceptors are primarily associated with vascular structures. The positive inotropic and chronotropic response of the heart is mediated by b-receptors. The neurotransmitters norepinephrine and EPI have a high affinity to these receptors. Adrenoreceptors are linked to a complex second-messenger system that modifies the signal transduction (40,41). The surface density of receptors can be up-regulated and down-regulated in response to the extraneuronal catecholamine concentration (42). This down-regulation of b-receptors, primarily by b1-receptors, has been described in patients with heart failure. In addition, the treatment of patients with heart failure today includes b-receptor antagonists, which have a beneficial effect on left ventricular function. This has led to an increasing interest in the noninvasive characterization of receptor distribution by tracer techniques.
The most commonly used tracer for postsynaptic imaging is [11C]-CGP12177, which represents a nonselective but hydrophilic b-receptor antagonist. Lipophilic tracers such as [11C]-propranolol and pindolol display a high retention in lung tissue and bind to internalized receptors. They are, therefore, not suitable for cardiac imaging purposes.
Delforge et al. (43) developed a quantitative imaging method that includes two tracer injections with varying specific activity, yielding absolute measurements of b-receptor density in the heart. Merlet et al. (11) applied receptor imaging to various disease groups including patients with dilated cardiomyopathy. This imaging approach has emerged as the most widely used test to visualize b-receptors. The radiochemistry of [11C]-CGP12177 is demanding because of the need for phosgene as a precursor for synthesis. More recently, [11C]-CGP12388 has been proposed as an alternative tracer with similar biologic characteristics, and it is easier to synthesize (44). Animal and clinical results are promising but await further evaluation (45,46).
Law et al. (47) reported the evaluation of [11C]-N-desmethyl-GB67 as a specific a1-receptor ligand in the heart. Rapid plasma clearance, no metabolites, and high myocardial retention yield good image quality. Future studies will have to document the ability of this new tracer to visualize and quantitate α-receptor density in the healthy and diseased heart.
Finally, radioactive-labeled ligands have been used to visualize the muscarinic receptors in the heart. There is a high density of these receptors in both the right and the left ventricles, which yields excellent image quality. This excellent imaging is somewhat surprising given the presence of only very sparse cholinergic presynaptic innervation of both cardiac ventricles. The PET research group at Orsay developed tracer approaches using [11C]-methylquinuclidinyl benzilate (MQNB) for the quantitative assessment of muscarinic receptors (48,49).
Imaging Protocols
An [11C]-HED PET imaging protocol typically includes dynamic PET acquisition for 40 to 60 minutes after injection and blood flow imaging using flow tracers such as nitrogen-13 ([13N])-ammonia or rubidium-82 (50,51,52,53,54,55). For [11C]-EPI PET imaging, with which high levels of [11C] metabolites are detected in the blood, correction for metabolite radioactivity in the arterial input function is required
for the calculation of tracer retention in the tissue (9). The use of [18F]-labeled tracers allows a longer imaging time, because of their longer physical half-life (110 minutes) compared with [11C] (20 minutes). The longer half-life of [18F] may obviate the necessity of an on-site cyclotron and thus may potentially allow for more widespread clinical use.
for the calculation of tracer retention in the tissue (9). The use of [18F]-labeled tracers allows a longer imaging time, because of their longer physical half-life (110 minutes) compared with [11C] (20 minutes). The longer half-life of [18F] may obviate the necessity of an on-site cyclotron and thus may potentially allow for more widespread clinical use.
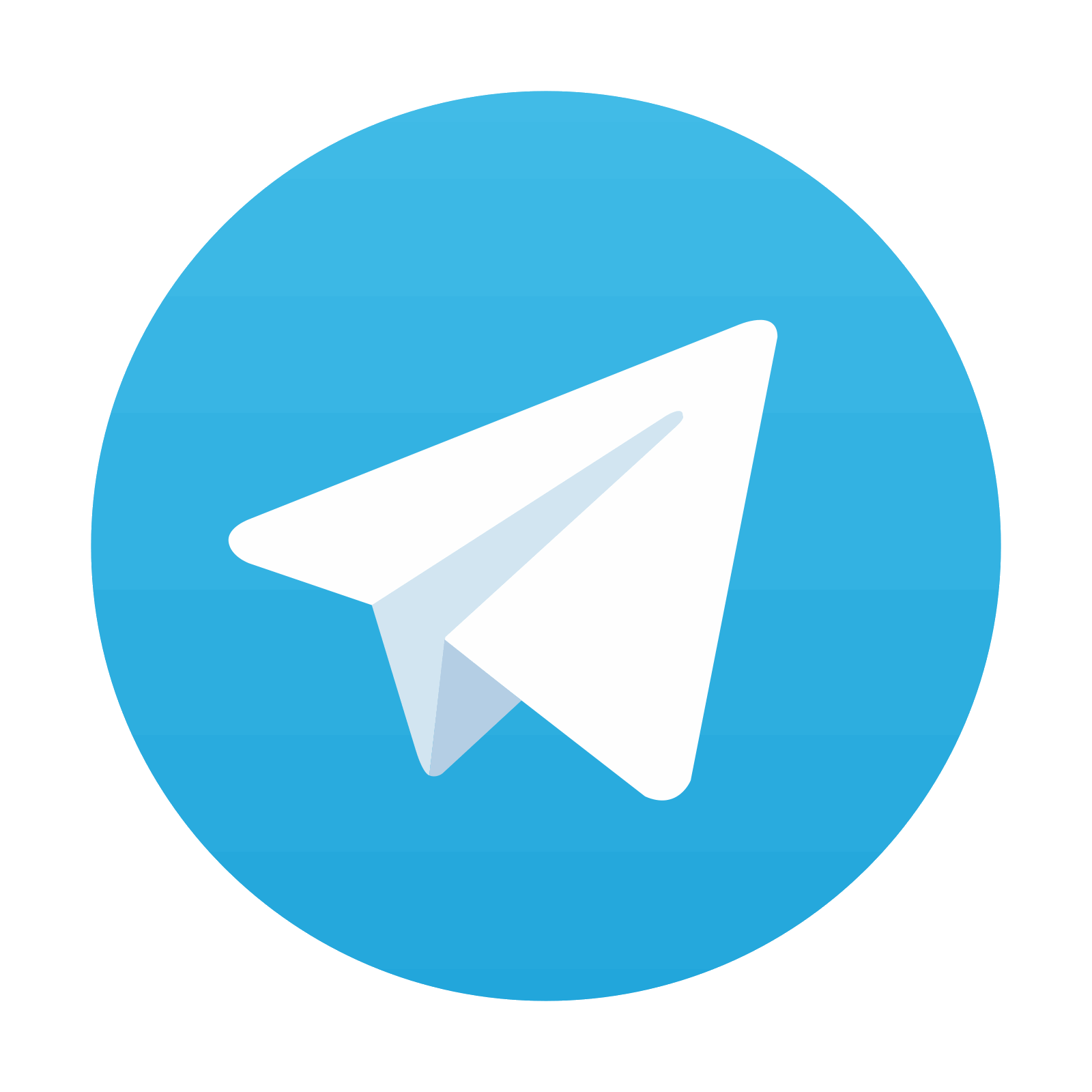
Stay updated, free articles. Join our Telegram channel
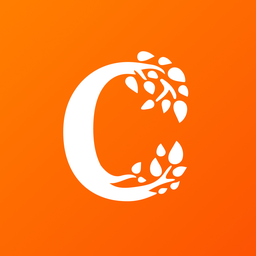
Full access? Get Clinical Tree
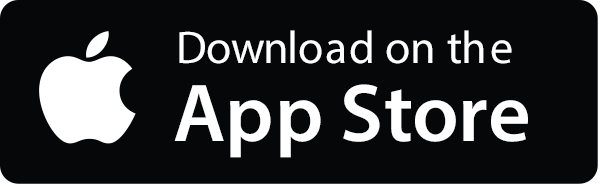
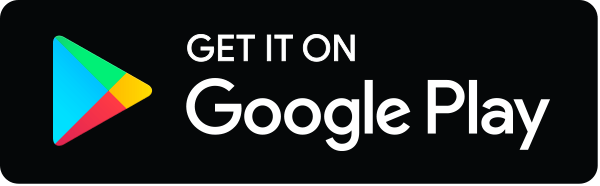