Myocardial Perfusion and Permeability
Michael Jerosch-Herold
Krishna Nayak
Tim Leiner
Rozemarijn Vliegenthart
Sven Plein
Vascular Supply of the Heart
Pathophysiology of Myocardial Ischemia
The heart is a metabolically highly active organ, which is reflected in its high basal oxygen consumption of 8 to 10 mL O2/min/100 g and high myocardial blood flow (approximately 50 to 200 mL/100 g/min at rest). Blood is delivered to the heart via the coronary arteries, which arise from the coronary sinuses of the aortic root. The main coronary arteries divide into smaller branches and subsequently into arterioles and capillaries. Importantly, myocardial blood flow (MBF) is not uniform across the heart; because of the higher contractility and shear stress in the subendocardium, this layer has the highest resting MBF and is most susceptible to ischemia. MBF also varies within the cardiac cycle; due to the forces of myocardial contraction, most MBF occurs in diastole.
MBF is mainly regulated by the resistance in precapillary arterioles and prearterioles, which maintain a near constant coronary blood flow over a wide range of diastolic pressures. These autoregulatory mechanisms also allow an increase of MBF in response to increased myocardial oxygen demand—up to fivefold in a healthy human. The difference between the resting MBF and the maximal MBF is the MBF reserve. Myocardial ischemia ensues when myocardial oxygen requirement exceeds oxygen supply (perfusion mismatch—note that this term should not be mistaken with the mismatch in brain perfusion where it relates to a mismatch between diffusion and perfusion), most commonly in the context of epicardial coronary stenosis (reducing MBF reserve) during physical exercise (increased oxygen demand). In a cascade of events, ischemia leads to metabolic derangements and contractile dysfunction before it manifests with electrocardiogram (ECG) changes and the typical symptom of angina (Fig. 70.1).1
When oxygen demand returns to baseline levels and the perfusion mismatch is resolved, for example, through the termination of exercise, ischemia and symptoms are typically relieved. Many other causes of increased oxygen demand or reduced oxygen supply are known (including left ventricular hypertrophy and anemia) and can cause or contribute to the development of myocardial ischemia. Ischemia usually affects the subendocardial layers before the mid- and subepicardium, reflecting the different metabolic rates and oxygen requirements between these layers (Fig. 70.2).
In the clinical setting, the detection of hypoperfusion during stress or the measurement of MBF reserve is used to determine the presence of significant, flow-limiting coronary artery disease that may benefit from revascularization. For this purpose, two principally different methods are available. First, ischemia can be provoked during physical or pharmacologic stress with agents such as dobutamine. Although perfusion imaging can in principle be performed during dobutamine stress, in clinical practice the high heart rates encountered and the potential for ischemia-induced complications (such as
ventricular arrhythmias) make this a challenging method for perfusion imaging. Alternatively, maximal MBF can be induced with pharmacologic vasodilator agents such as adenosine. This leads to a lower heart rate increase and is the most commonly used clinical stress mode. Vasodilator stress does not typically induce ischemia, but the presence of reduced MBF at stress or a reduced MBF reserve is considered as a possible consequence of significant coronary artery stenosis.
ventricular arrhythmias) make this a challenging method for perfusion imaging. Alternatively, maximal MBF can be induced with pharmacologic vasodilator agents such as adenosine. This leads to a lower heart rate increase and is the most commonly used clinical stress mode. Vasodilator stress does not typically induce ischemia, but the presence of reduced MBF at stress or a reduced MBF reserve is considered as a possible consequence of significant coronary artery stenosis.
Pathophysiology of Myocardial Infarction
Acute myocardial infarction can have several causes, as summarized in the 2012 universal definition of myocardial infarction (Table 70.1).2
Acute myocardial ischemia, regardless of its causative mechanism, triggers a complex metabolic and ultrastructural response that leads to edema, metabolic derangements, contractile arrest, and ultimately myocyte necrosis. These processes initially affect the more vulnerable subendocardial layers and subsequently progress in a “wavefront of ischemia” to the outer myocardial layers.3 The final extent of necrosis depends on numerous factors, including the myocardial volume subtended by the culprit coronary artery, the presence and extent of collateralization, and the duration of ischemia. Restoration of myocardial blood flow can occur spontaneously or be achieved with the use of thrombolysis or percutaneous coronary intervention. Although timely intervention limits the extent of myocardial infarction (MI), abrupt reperfusion of injured myocardium can also cause further damage, referred to as reperfusion injury. This can lead to microvascular obstruction (MO) or hemorrhagic transformation in the infarct core.
TABLE 70.1 UNIVERSAL DEFINITION of MYOCARDIAL INFARCTION | ||||||||||||||
---|---|---|---|---|---|---|---|---|---|---|---|---|---|---|
|
Following acute MI, restorative processes lead to replacement of necrotic myocytes with fibrous scar, typically associated with thinning of the affected myocardial area. In the peri-infarct zone, which has been transiently ischemic but where necrosis has not occurred, transient contractile dysfunction (“stunning”) can occur and typically resolve over time.4 Soon after the acute event, the heart, including myocardium remote from the infarct zone, undergoes adaptive processes that are classified as “remodeling.”5
Practical Considerations for Stress Testing in Magnetic Resonance Imaging and Computed Tomography
Setup
The setup for a stress magnetic resonance imaging (MRI) or computed tomography (CT) study differs in several ways from that for a nonstress examination because of the need to administer a stress agent and to monitor its effects.
Patient Preparation
Appropriate patient information about the procedure is mandatory prior to performing a stress MRI or CT study. In addition, patients need to be screened for contraindications to stress medication, contrast agents, x-ray exposure (CT) or exposure to magnetic fields (MRI). Because caffeine is a competitive antagonist of vasodilator stress agents, patients are instructed not to consume any caffeine-containing food or beverage for 24 hours before the study. It is also generally recommended to withhold cardiac medication, in particular vasodilators, β-blockers and calcium channel antagonists 24 to 48 hours before a stress study. For perfusion studies, patients require ideally two intravenous lines to deliver the stress and contrast agents separately, although some centers deliver both agents through a single line using a short Y-shaped connector. At least one of the intravenous lines should be placed in a large antecubital vein for administration of the contrast agent. If regadenoson is used as the stress agent, only one line is required as this agent is injected as single bolus a couple of minutes before the perfusion scan.
Equipment
Most of the hardware required for MRI stress testing is part of the standard equipment available in any radiology department performing cardiac MRI. For CT, at least ECG gating and monitoring are essential and can be performed with a specialized unit or standard manufacturer-supplied ECG monitoring equipment. As described later, different techniques exist for evaluation of myocardial perfusion with CT. For static CT, 64-multidetector CT and higher generations can be used. For dynamic CT, the newest generation CT scanners are needed, such as
second-generation dual source CT or 256/320-multidetector CT. Blood pressure should be monitored with an automated system at 1-minute intervals during the administration of stress agents and until symptoms have resolved and hemodynamic parameters normalized. With CT, stress agents are administered via standard medical infusion pumps. For MRI studies, appropriate MR-compatible monitoring equipment and infusion pumps should be used. Alternatively, non-MR-compatible equipment located outside the scanner room can be used with long intravenous lines fed to the patient via the scanner’s waveguide (i.e., the conduits in the wall that allow access through the radiofrequency [RF] shield that covers the entire magnet suite). Finally, for all perfusion imaging, an automated injection pump is recommended to deliver a reproducible and rapid contrast agent bolus.
second-generation dual source CT or 256/320-multidetector CT. Blood pressure should be monitored with an automated system at 1-minute intervals during the administration of stress agents and until symptoms have resolved and hemodynamic parameters normalized. With CT, stress agents are administered via standard medical infusion pumps. For MRI studies, appropriate MR-compatible monitoring equipment and infusion pumps should be used. Alternatively, non-MR-compatible equipment located outside the scanner room can be used with long intravenous lines fed to the patient via the scanner’s waveguide (i.e., the conduits in the wall that allow access through the radiofrequency [RF] shield that covers the entire magnet suite). Finally, for all perfusion imaging, an automated injection pump is recommended to deliver a reproducible and rapid contrast agent bolus.
Safety
Performing pharmacologic stress testing within the environment of a CT or MRI scanner requires particular safety consideration. Although rare, the agents used for stress testing can have serious and potentially life-threatening side effects. The ability to respond rapidly and adequately to any arising complications must be considered in the local setup. Staff need to be appropriately trained in the procedures to provide basic and advanced life support, which should have been rehearsed (i.e., how to remove patients from the scanner to an environment where resuscitation procedures can be safely performed). It is often recommended that such evacuation procedures should not take more than 1 minute. This is particularly pertinent for stress examinations in an MRI scanner, where the magnetic field prevents the use of defibrillators and rapid access of medical teams. Access to emergency medications and a resuscitation trolley needs to be ensured.
Vasodilators are by far the most commonly used pharmacologic stress agents in CT and MRI, with adenosine, dipyridamole, and regadenoson in clinical use. These agents can cause atrioventricular block, bronchospasm, and hemodynamic complications and are, therefore, contraindicated in several conditions (Table 70.1). Inotropic stress is less frequently performed. The stress agent used is dobutamine, and its most important side effect is the potential induction of ventricular arrhythmias (Table 70.2).
TABLE 70.2 CONTRAINDICATIONS TO VASODILATOR AND DOBUTAMINE STRESS TESTING | ||
---|---|---|
|
Echocardiogram
Reliable and continuous ECG monitoring is essential for stress examinations. In MRI, this can be challenging because the magnetic field distorts the ECG signal. The ECG, therefore, cannot be used to detect ischemic changes such as ST segment depression. However, it is valuable to monitor the heart rate and detect a high-grade atrioventricular block.
Dealing with Challenging Patients
Poor Echocardiogram
A reliable ECG is essential for a high-quality MRI or CT perfusion study. Care needs to be taken to obtain a good ECG prior to the perfusion study being commenced. Repositioning of ECG leads may be required, particularly in the MRI environment, where the magneto-hydrodynamiceffect can distort the appearance of the ECG. All vendors have developed reliable ECGs and offer algorithms for the best placement of the ECG electrodes with their scanners. In the rare instances where a good ECG cannot be obtained, it is in principle possible to trigger the acquisition from the patient’s pulse, although this is rarely required in clinical practice.
Arrhythmia
Most MRI perfusion pulse sequences are set up to permit acquisition at heart rates up to 100 to 120 beats per minute. Beyond this rate, acquisition usually defaults to every second R-R interval. Although this may be sufficient for visual analysis, it reduces the reliability of quantitative estimates of MBF and should be avoided. Patients with sinus tachycardia or tachyarrhythmia, therefore, pose a challenge for perfusion MRI. Rate-controlled atrial fibrillation is less of a challenge as long as each R-R interval is reasonably constant. Indeed, because perfusion MRI is a dynamic acquisition in which a full image is acquired for every slice in every heart beat, it is often less affected by arrhythmia than other MRI methods in which data are averaged over several heart beats such as cine imaging. However, arrhythmia can lead to variable R-R intervals and thus inconsistent timing of acquired images in the cardiac cycle, which can make image interpretation difficult. Patients with poor breath-hold ability are another challenging patient group as images can be affected by respiratory motion artifacts.
Geek Box 1: Acquisition Protocols for Stress MR and CT
Adenosine stress myocardial perfusion MRI:
Prepare patient as outlined above
Define left ventricular short axis (SAX) from scout images
Perform a test run of the perfusion pulse sequence without contrast
Start vasodilator at the following dose: adenosine (0.14 mg/kg/min IV for 3 to 4 minutes), dipyridamole (0.57 mg/kg, up to 60 mg IV over 4 minutes) or regadenoson (0.4 mg bolus injection)
Monitor heart rate and blood pressure
At peak hyperemia, start data acquisition (at least 40 to 50 dynamics)
Inject gadolinium-based contrast medium (0.025 to 0.1 mmol/kg bodyweight at a rate of 3 to 7 mL/sec, followed by a 20-mL flush of normal saline)
Give breathing instructions, so that patient holds breath just before contrast arrives in left ventricle
Stop stress agent and continue to monitor patient until symptoms have relieved and hemodynamics are normalized again
Side effects and complications of adenosine infusion are usually rapidly reversible on stopping the infusion. If required, persistent side effects or complications of adenosine can be treated with intravenous aminophylline.
Dobutamine stress MRI:
Prepare patient as outlined above
Define left SAX and two orthogonal long axes from scout images
Acquire resting cine images in three SAX and two long axis planes
Start dobutamine at 5 to 10 mcg/kg/min and increase dose in 2- to 3-minute intervals and increments of 5 to 10 mcg/kg/min to a maximum of 30 to 40 mcg/kg/min. If the target heart rate of 85% of the maximal heart rate predicted for age cannot be reached, up to 2 mg atropine can be administered at incremental doses.
Monitor heart rate and blood pressure at each increment
Repeat acquisition of cine images in three SAX and two long axis planes at each increment
Consider performing stress perfusion study at peak stress dose
Stop stress agent and continue to monitor patient until symptoms have relieved and hemodynamics are normalized again
Criteria for early termination of the test are severe chest pain, significant ventricular or supraventricular arrhythmia, severe hypertension or blood pressure drop, and any intolerable side effects
Side effects of dobutamine that do not resolve after termination of the infusion can be reverted by metoprolol (1 to 5 mg) or esmolol intravenously.
Adenosine perfusion CT:
The use of CT for myocardial perfusion imaging is still in the research phase and protocols are less well standardized than for cardiovascular magnetic resonance. Several different protocols have been proposed, depending on the type of scanner. The main distinction in protocols is between a static and dynamic approach to evaluate myocardial blood supply (see below for details). Example protocol for dynamic perfusion CT:
Prepare patient as outlined above with two IV lines
Plan acquisition to cover heart from pulmonary artery to apex
Plan a timed-bolus or bolus tracking acquisition
Start vasodilator at the following dose: adenosine (0.14 mg/kg/min IV for 3 to 4 minutes), dipyridamole (0.57 mg/kg, up to 60 mg IV over 4 minutes), or regadenoson (0.4 mg bolus injection)
Monitor heart rate and blood pressure
At peak hyperemia, start data acquisition and instruct patient to hold the breath
Inject iodine-based contrast medium (40 to 60 mL at a rate of 3 to 7 mL/sec, followed by a 60-mL flush of mixed contrast agent/normal saline)
Stop stress agent and continue to monitor patient until symptoms have passed and hemodynamics are normalized again.
Similar challenges exist for CT perfusion. Although a regular, low heart rate is less essential for CT imaging of the myocardium compared with imaging of the coronary arteries, motion artifacts in myocardial contour can occur with arrhythmia. This could affect the accuracy of quantitative perfusion analysis.
Complications during Stress
Patients are frequently symptomatic during vasodilator stress and complain of shortness of breath, flushing, and chest discomfort. These symptoms are normal and patients should be reassured. Most side effects are also
quickly reversible upon termination of the stress agent. Serious complications during vasodilator stress are rare but do occur. Patients may suffer respiratory complications, especially bronchospasm. If considered clinically significant, respiratory complications require termination of the stressor, application of bronchodilators, and, occasionally, treatment with intravenous aminophylline, which is an adenosine antagonist. Atrioventricular block may occur but is usually transient and, in asymptomatic patients, can be monitored. Persistent or symptomatic arteriovenous block should be treated by stopping the stressor and, if required, by the administration of intravenous aminophylline. Blood pressure needs to be monitored throughout the stress study and a significant and persistent drop (>20 mm Hg) should lead to termination of the stressor.
quickly reversible upon termination of the stress agent. Serious complications during vasodilator stress are rare but do occur. Patients may suffer respiratory complications, especially bronchospasm. If considered clinically significant, respiratory complications require termination of the stressor, application of bronchodilators, and, occasionally, treatment with intravenous aminophylline, which is an adenosine antagonist. Atrioventricular block may occur but is usually transient and, in asymptomatic patients, can be monitored. Persistent or symptomatic arteriovenous block should be treated by stopping the stressor and, if required, by the administration of intravenous aminophylline. Blood pressure needs to be monitored throughout the stress study and a significant and persistent drop (>20 mm Hg) should lead to termination of the stressor.
Methods for Contrast-Enhanced Myocardial Perfusion Magnetic Resonance Imaging and Computed Tomography
Principles and Pulse Sequences
Myocardial perfusion MRI relies on detecting the effects of an injected contrast agent on the signal from 1H nuclei (e.g., from water in tissue or blood, or the 1H nuclei in lipids). MRI contrast agents, such as the commonly used gadolinium chelates, cause a reduction of T1, and T2 or T2* relaxation times. Specifically, the inverse of T1 or T2* relaxation times, the relaxation rates R1 (= 1/T1), or R2* (= 1/T2*), are in principle proportional to the concentration of contrast in blood or tissue. Myocardial perfusion MRI is based on acquiring images with the signal intensity rendered sensitive to T1 or T2*, thereby reflecting the local concentration of contrast agent.
Though T2*-weighting imaging was used initially in some studies for of myocardial perfusion, T1 weighting is today the predominant method for detection of contrast in the heart. T1-weighted perfusion MRI, when compared with T2*-weighted techniques, is less susceptible to cardiac motion, mostly by virtue of the short(er) echo times used for T1-weighted imaging. T2* weighting, in particular at higher field strengths (> ∼1T) causes signal loss that extends beyond the space permeated by the contrast material.
T1 weighting in perfusion MRI is enhanced (beyond the T1 weighting already achieved by the image readout) by applying a so-called magnetization preparation before the image readout, which can be in the form of a magnetization inversion pulse or a magnetization saturation pulse. A saturation recovery preparation has become the method of choice because it renders the signal intensity insensitive to the repetition time between applications of the saturation preparation, as signal is saturated at every R-R interval using ECG triggering and, therefore, is insensitive to the patient’s heart rate. The image readout itself also imparts T1 weighting to the acquired signal (e.g., by using short repetition and echo times for gradient-echo imaging), but if it is preceded by magnetization preparation, the T1 weighting can be strengthened.
MRI techniques used for image readout in perfusion MRI studies need to offer short acquisition times, so that several image sections can be acquired in each R-R interval and they need to be predominantly T1 weighed to generate a high signal: single-shot gradient-echo imaging, with and without steady-state free precession, hybrid gradient-echo, and echo-planar imaging are common choices, listed here in the approximate order of decreasing frequency of usage.
Field strength is an important consideration in the choice of an optimal technique: steady-state free precession techniques are not used for myocardial perfusion MRI at 3T or higher because of their sensitivity to frequency offsets, which can be induced by the transit of the contrast bolus. Table 70.1 lists the most common MRI perfusion techniques.

Tips and tricks for MRI perfusion image acquisition:
Give breath-hold instructions to avoid respiratory motion during the first pass of contrast. The perfusion scan is generally too long for breath-hold throughout the entire scan.
Use a power-injector with an injection rate of 3 to 4 mL/min to optimize the sensitivity of the contrast enhancement to myocardial perfusion (rather than contrast delivery being the bottle neck).
If dark lines (dark banding artifact due to susceptibility or Gibbs ringing) are observed along the endocardial border, then ideally a higher spatial resolution in the phase-encoding direction should be used, and/or the echo time should be reduced, if possible. This may not be feasible in clinical practice.
If the image acquisition is not repeated every heart beat, despite ECG-trigger and regular heart beat, the image acquisition duration should be reduced or a slice should be dropped from the acquisition. Test this with a dry run.
At the time of writing, myocardial perfusion MRI still relies predominantly on two-dimensional, multislice image acquisitions to cover the heart. Typically three or four slices can be imaged during each heart beat, with the image acquisition in each slice taking approximately 200 ms or less to freeze cardiac motion. By necessity, the acquisition of the images for each slice is spread over the cardiac cycle. Parallel acquisition of image data with a distributed coil array is crucial for optimizing multislice coverage of the heart to keep image acquisition as
short as possible and thereby minimize image artifacts. Additionally, sparse sampling (along the temporal domain) can be used for the same purpose. This basically means that varying subsets of data are acquired for each dynamic view in a perfusion study, and the missing data are inferred from “training data” with lower spatial resolution or other techniques for sparse sampling (e.g., compressive sensing, temporal filtering, or use of a composite reference image in the HYPR [HighlY constrained back PRojection] technique). The feasibility of three-dimensional image acquisitions covering the heart without interslice gaps has been demonstrated, using very high (greater than or approximately × 10) image acquisition acceleration factors.
short as possible and thereby minimize image artifacts. Additionally, sparse sampling (along the temporal domain) can be used for the same purpose. This basically means that varying subsets of data are acquired for each dynamic view in a perfusion study, and the missing data are inferred from “training data” with lower spatial resolution or other techniques for sparse sampling (e.g., compressive sensing, temporal filtering, or use of a composite reference image in the HYPR [HighlY constrained back PRojection] technique). The feasibility of three-dimensional image acquisitions covering the heart without interslice gaps has been demonstrated, using very high (greater than or approximately × 10) image acquisition acceleration factors.
CT assessment of the myocardium is based on the same principles as MRI evaluation because contrast kinetics are comparable for iodine contrast and gadolinium contrast. For the CT-based assessment of MBF, two different strategies need careful distinction: a static and dynamic image acquisition approach.6 In the static approach, a snapshot image is acquired of contrast distribution over the myocardium. This reflects the myocardial blood supply at one point in time. In many publications, this is referred to as perfusion imaging, but actually this strategy does not allow for quantification of perfusion parameters such as MBF or upslope of the signal intensity profile. The main evaluation is based on visual detection of hypoperfused myocardial segments that can show as hypoattenuating areas during the first pass of iodine contrast through the left ventricle. The background of this finding is that myocardial segments with reduced blood supply have a reduced delivery of contrast material and thus a lower CT density compared with surrounding myocardial segments with normal perfusion. Often, the common CT coronary angiography scan is used for this evaluation.
Geek Box 2: Key Requirements for Pulse Sequence Design in Stress MR and CT
Dynamic image acquisition by MRI:
Use a protocol for fast, multislice dynamic imaging with strong T1 weighting.
Image acquisition should be ECG triggered, so that at every slice location each dynamic scan is acquired during the same phase of the cardiac cycle for approx. 60 heart beats.
T1 weighting should include magnetization preparation before image readouts (e.g., with magnetization saturation), followed by the image acquisition during magnetization recovery.
To obtain uniform T1 weighting for all slices, the magnetization preparation should be repeated for each slice.
Minimize the sensitivity of the T1 weighting in the ventricular cavity to inflow effects by using a nonslice-selective magnetization preparation. This is particularly important if the arterial contrast enhancement is used in the postprocessing analysis.
Use parallel imaging and/or temporal undersampling to minimize the two-dimensional image acquisition duration for each slice, as this reduces artifacts from cardiac motion.
Dynamic image acquisition by CT:
CT scan protocols differ by strategy (static vs. dynamic image acquisition) and by vendor. An example CT protocol for dynamic image acquisition of myocardial blood supply for the dual-source CT scanner is:
Scan parameters: 100 kV, 300 mAs.
A test bolus is used to evaluate the best timing to start image acquisition.
50 mL iodine contrast is injected, followed by 50 mL of saline (flow rates 6 mL/sec).
The patient is asked to take a deep breath and hold breath for the duration of the scan.
The acquisition is initiated 4 seconds before the arrival of the first iodine contrast.
Data acquisition is performed under ECG-triggering, at every other R-R interval.
Image acquisition takes place during end systole (250 ms after the R-wave peak).
The scan table shuttles back and forth between two positions between the acquisitions.
Complete datasets of the cardiac volume are acquired approximately every 2.8 seconds.
Standardized acquisition time is 30 seconds.
The protocol for adenosine stress scanning has been described in the text.
Alternatively, a second scan acquisition can be performed under adenosine infusion to assess myocardial segments with reduced blood supply under stress. In principle, the static approach is feasible with all CT scanners that can be used for CT coronary angiography. Dedicated postprocessing software can make the hypoperfused myocardial segments more easily detectable, for example, by providing color maps of CT attenuation. In static CT imaging for evaluation of MBF, correct bolus timing is essential. Beam-hardening artifacts at the border of endocardium and a contrast-filled left ventricle can negatively
influence assessment. A variation of the static approach is image acquisition and image reconstruction of more than one energy x-ray spectrum. This approach has been primarily evaluated with dual-source CT technology.7
influence assessment. A variation of the static approach is image acquisition and image reconstruction of more than one energy x-ray spectrum. This approach has been primarily evaluated with dual-source CT technology.7
With the second strategy, the dynamic approach, quantification of perfusion parameters are indeed possible. In dynamic CT acquisition, myocardial perfusion is assessed during the passage of a contrast bolus through the left ventricular myocardium. Images are acquired at multiple time points (time resolved). Larger detector width, such as in 320-multidetector CT technology, and quick back-and-forth shuttling of the scanner table in several other CT models allow for dynamic perfusion imaging of most or all of the heart. Quantitative evaluation of myocardial perfusion is deemed of importance to assess the hemodynamic significance of a coronary stenosis. Currently, only positron emission tomography (PET) is also capable of providing this information. Cardiac MRI is less applicable for this purpose due to the nonlinear relationship between signal intensity after injection of gadolinium-based contrast agents and tissue perfusion. With iodine-based CT contrast agents, this relationship is linear.8 The drawbacks of dynamic CT perfusion include a relatively high radiation exposure (10 to 18 mSv, depending on technique) and the fact that usually a separate scan acquisition is needed for coronary artery analysis.
Methods for Noncontrast Myocardial Perfusion Magnetic Resonance Imaging
This section describes two emerging MRI methods for the assessment of myocardial ischemia without any contrast agents. They utilize endogenous contrast, which is based on blood flow and blood oxygenation, respectively, and can, therefore, be performed safely and repeatedly in humans. The data so far produced with these methods is poorer than that of first-pass MRI in terms of spatial resolution, coverage, and signal-to-noise ratio. However, they can be applied broadly, including for patients with heart failure, chronic kidney disease, and even end-stage renal disease, where gadolinium-based agents would be contraindicated. The acquisition and postprocessing techniques for these two techniques continue to evolve; however, both have produced promising results in single-center clinical studies.
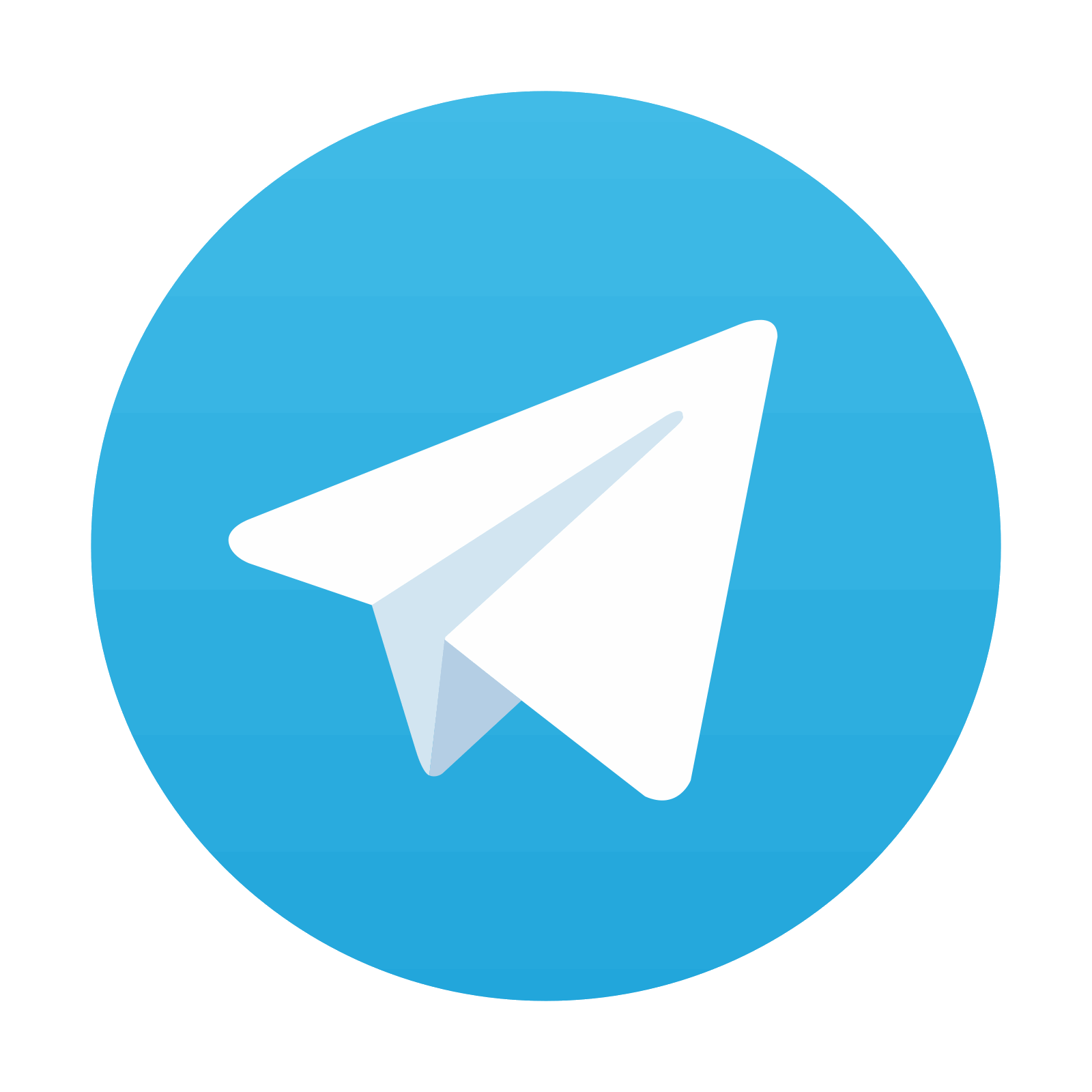
Stay updated, free articles. Join our Telegram channel
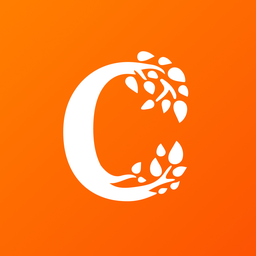
Full access? Get Clinical Tree
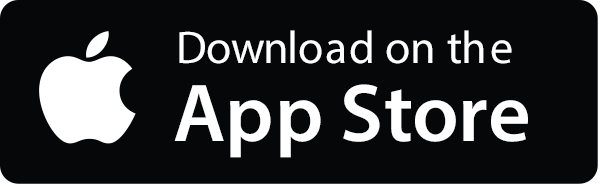
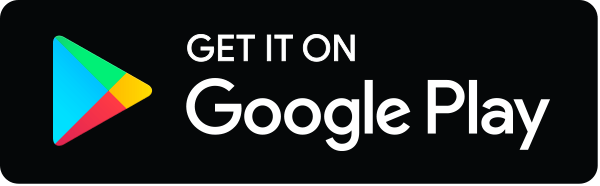