Neurosurgical diagnosis and intervention has evolved through improved neuroimaging, allowing better visualization of anatomy and pathology. This article discusses the various systems that have been designed over the last decade to meet the requirements of neurosurgical patients and opines on the potential future developments in the technology and application of intraoperative MRI. Because the greatest amount of experience with intraoperative MRI comes from its use in brain tumor resection, this article focuses on the origins of intraoperative MRI in relation to this field.
Successful neurosurgical procedures hinge on the accurate targeting of regions of interest. Resection of brain tumors is enhanced by the surgeon’s ability to accurately define margins. Epileptic foci are identified by coregistration of functional and antatomic information, and stereotactic targets must be pinpointed with submillimetric accuracy for surgical efficacy. Specialized neuronavigational tools have been developed over the last 20 years to assist surgeons in these endeavors; the development of MRI-guided navigation systems represents a significant improvement in the surgical treatment of various intracranial lesions. The ability for most intraoperative image guidance systems to remain faithful to the anatomy once the cranium has been opened remains problematic, however. “Brain shift,” the term applied to the dynamic change that intracranial anatomy undergoes after craniotomy, burr hole placement, drainage of cerebrospinal fluid, or resection of a lesion, compromises the localization of neural structures in space relative to where they were when preoperative images were acquired ( Fig. 1 ). Gliomas also pose a particular challenge to surgeons because many of these tumors (particularly low-grade gliomas) do not possess distinct capsules. As a result, even well-trained human eyes are incapable of discerning where the border of the lesion ends and viable brain begins. This uncertainty leads to two problems: (1) inadequate resection secondary to the surgeon stopping at what appears to be grossly abnormal tissue (so as to avoid neurologic damage) and (2) neurologic damage caused by aggressive surgery in which resection ends only when clearly normal brain tissue is visualized.
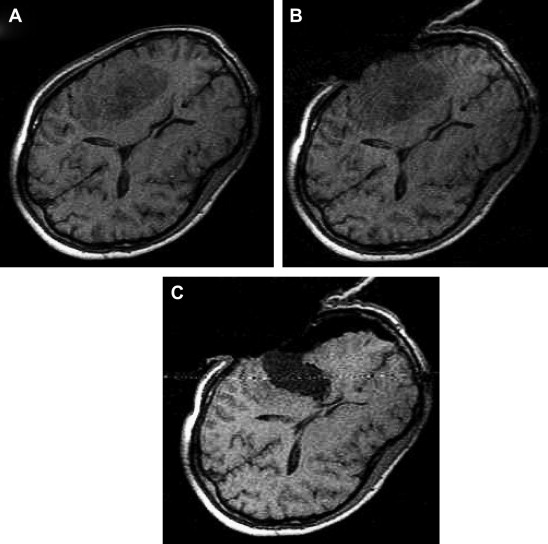
Only intraoperatively acquired images can provide neurosurgeons with the information needed to perform real-time, image-guided surgery. Uncertainty is reduced significantly when the surgeon places an instrument at the edge of what is believed to be the resection cavity, and a small nodule of tumor is immediately identified by intraoperative imaging. Avoidance and preservation of eloquent cortex such as motor, speech, and visual areas depend on precise identification of these regions during the procedure. The boundary between tumor and viable neural tissue is often difficult to see with the naked eye, so the superimposition of functional MRI, diffusion tensor imaging, and awake cortical mapping images eliminates a surgeon’s uncertainty in determining tumor boundary and shifting brain structures. This leads to surgeons achieving maximal lesion resection while minimizing untoward neurologic sequelae. Maximal lesion resection is a principal goal in tumor resection because abundant evidence indicates that a more complete resection directly impacts the survival time of patients with low- and high-grade gliomas.
Origins of intraoperative MRI: 0.5T open-configuration prototype
The origin of iMRI for neurosurgery was the Magnetic Resonance Therapy (MRT) Unit at Brigham and Women’s Hospital (BWH) in Boston, Massachusetts. It began as a collaborative project among four groups: Ferenc Jolesz of the Department of Radiology at BWH, engineers at General Electric Medical Systems (Milwaukee, Wisconsin), the neurosurgical service at BWH with Dr. Peter Black as head, and the department of otorhinolaryngology with Marvin Fried as director. Throughout the late 1980s, these physicians and scientists collaborated in the development of an open-configuration MRI scanner that allowed surgery to be performed with concurrent intraoperative image guidance. At the time of inception, the closed-configuration of conventional MRI systems precluded direct access to the patient; therefore, fundamental changes in magnet and coil design and display methods were necessary to fully realize the concept of iMRI. This concept was a radical departure from MR physics of the time, with the magnetic field highest in the space between the double donut.
Early interventional procedures in an open MRI system were performed in a low-field imager by Gronemeyer and colleagues. This system provided access to patients through a horizontal gap in its magnet. Access was significantly limited, however, and open surgeries that required full access to patients were impractical. Based on this information, after discussing several alternative designs, a “double donut” magnet system that would allow free access to patients within the magnetic field was chosen by BWH for development. The initial research and development phase came to fruition in 1994 with the completion and installation of a prototype midfield intraoperative MRI system (GE 0.5-T Signa SP) unit at the BWH ( Fig. 2 ).
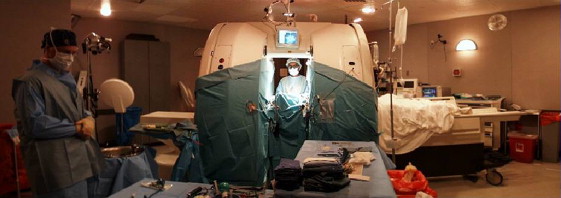
Direct access to patients was achieved by the construction of two vertically oriented superconducting magnets with coils in separate but communicating cryocoolers. This design results in a vertical gap between the coils through which patients can be fully accessed during image acquisition. Niobium tin, which has a maximum superconducting transition at higher temperatures than the more common niobium titanium, allows for sufficient cooling of the coils and thermal shield with cryocooler assembly, which eliminates the need for liquid helium coolant. This design resulted in a significantly increased area of patient access: the modified magnet provides a spherical imaging volume 30 cm in diameter and a 56-cm wide area of patient access, allowing surgeons and first assistants to be positioned on either side of patients. In addition to the wide patient access area, the configuration of the “double-donut” magnet allows the position of patients within the imager to be flexible; the table can be inserted into the magnet along two orthogonal axes (“end docked” and “side docked”), which allows convenient access to different areas of anatomy. Because of the open configuration of the MRT, surgeons can perform various percutaneous, interventional, endoscopic, or open surgical procedures while standing or sitting and simultaneously viewing intraoperatively obtained MRI displayed on monitors placed in the gap of the magnet.
Many challenges needed to be met during the original implementation of iMRI, including the development of MRI-compatible equipment, instruments, and various tools along with the integration of the intraoperative display of images, the audiovisual communication among the team members, and the interactive manipulation of image data.
The initial phase of the iMRI project was slowed down by the unavailability of MR-compatible surgical instruments. In many cases, extensive changes were required to adapt instruments and equipment to the unique electromagnetic environment. Many ferromagnetic surgical instruments were replaced by titanium, providing the essential capabilities required for craniotomies without becoming a ballistic hazard. Several metallic instruments that were not ferromagnetic still caused a substantial artifact when placed near a target within the imaging field of view and could not be used. An early problem was the headholder, which had to be firm, nonferromagnetic, and flexible. It was possible to create a headholder similar to the Mayfield device made of high performance plastic, but it took many months. The next challenge was the power drill; for more than a year, the only procedures that could be done were biopsies because there was no way to turn a craniotomy flap. The Midas Rex Corporation (Medtronic, Minniapolis, Minnesota) finally was able to create a nonferromagnetic drill. The operating microscope was the third major device to be created; it was possible to create a plastic microscope with nonferromagnetic joints that, although simple, was adequate. Finally, we were able to develop a bipolar coagulator whose current would not interfere with the magnetic field. Each of these technologic developments took 6 to 12 months to complete, but gradually it was possible to do surgery in the intraoperative GE Signa system just as readily as in a routine operating room. Anesthesia and patient monitoring systems that did not emit any electronic noise and could function during a scan were developed and installed within the MRT suite ; fortunately, they had been created for performing pediatric MR imaging under anesthesia.
The development of imaging during surgery led to the “Surgical Planning Laboratory” at BWH. The occurrence of surgically induced volumetric deformations known as brain shift has been well established. There were no detailed analyses, however, of the changes that occur during surgery. As a result, Gering and colleagues at BWH developed a volumetric display software (3D Slicer; www.slicer.org ) that allowed quantitative analysis of the degree and direction of brain shift ( Fig. 3 ). For 25 patients, multiple intraoperative volumetric image acquisitions were extensively evaluated. It was found that brain shift is a continuous dynamic process that evolves differently in distinct brain regions. The authors concluded that only serial imaging or continuous data acquisition can provide consistently accurate image guidance. Further refinements in tracking intraoperative brain deformation were performed in a pilot study by Archip and colleagues in 2008.
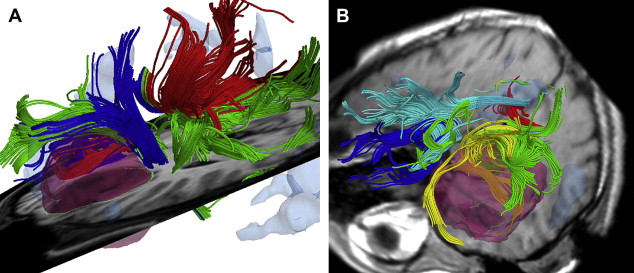
The combination of the 0.5-T iMRI and three-dimensional slicer transformed the MRT into an exceptionally effective tool for neurosurgeons. Since the first craniotomy for brain tumor resection in 1996, more than 1000 craniotomies for intracranial tumor resection have taken place in the MRT (40% low-grade gliomas, 50% high-grade gliomas, and 10% other intracranial lesions such as metastases, meningiomas, and vascular malformations). In most patients with brain tumor operated on in the MRT, resection rates of 80% or more were achieved. This percentage reinforces the value of iMRI in extension of lifespan of patients, because patients with subtotal tumor resection are at a higher risk of recurrence and death compared with patients with gross total tumor removal.
Expanding the scope of open-configuration intraoperative MRI
The BWH MRT was the original iMRI system but it was by no means the last. Because of the specialized nature of the equipment, the entire MRT suite needed to be custom-made to accommodate the scanner. Room shielding, coolant, and power consumption were only a few of the expensive and high-maintenance aspects of the MRT. iMRI had proven itself to be a powerful tool in the hands of neurosurgeons attempting to achieve maximal tumor resection while preserving neurologic function, but how could institutions and practitioners take advantage of this technology without embarking on the expense of a major remodeling of their operative suite?
There were several answers, all driven by neurosurgeons. A major center was Erlangen, where Rudolph Fahlbusch helped to develop multiple concepts of Siemens for intraoperative imaging. This system moved from a side-opening low field to a system in which a table rotated into and out of a 1.5-T closed-bore magnet. Fahlbusch showed that for pituitary tumors and low-grade gliomas this system had a major advantage over other systems. A second answer was driven by the Israeli surgeon Moshe Hadani and his group. Initially introduced in 2001 by Hadani and collagues, the PoleStar (Medtronic Navigation, Louisville, Colorado) N-10 iMRI offered an open-configuration, portable 0.12-T magnet that required only modest remodeling of the operative suite. The device was stored in what amounted to a small garage within the operating theater. The N-10’s compact size and low magnetic footprint allowed units to be integrated into multiple institutions’ conventional operating rooms. Despite a slightly increased time for induction of anesthesia and intubation, the units made a significant and positive impact on the safety and completeness of tumor resection in adult and pediatric intracranial procedures. PoleStar recently introduced a higher-field (0.15-T) N-20 ( Fig. 4 ), and initial evaluations confirm that the accuracy, versatility, and quality of this new-generation iMRI scanner are at least as good as the N-10. Clearly further clinical analysis of the accuracy on clinical cases using the N-20 is needed to confirm that these results will bear out in surgical reality.
Other open-configuration iMRIs have since been developed. A 0.3-T horizontal iMRI by Hitachi at the University of Cincinnati is a customized diagnostic iMRI, but the draping configuration does not lend itself to the sterile nature of neurosurgical interventions.
Expanding the scope of open-configuration intraoperative MRI
The BWH MRT was the original iMRI system but it was by no means the last. Because of the specialized nature of the equipment, the entire MRT suite needed to be custom-made to accommodate the scanner. Room shielding, coolant, and power consumption were only a few of the expensive and high-maintenance aspects of the MRT. iMRI had proven itself to be a powerful tool in the hands of neurosurgeons attempting to achieve maximal tumor resection while preserving neurologic function, but how could institutions and practitioners take advantage of this technology without embarking on the expense of a major remodeling of their operative suite?
There were several answers, all driven by neurosurgeons. A major center was Erlangen, where Rudolph Fahlbusch helped to develop multiple concepts of Siemens for intraoperative imaging. This system moved from a side-opening low field to a system in which a table rotated into and out of a 1.5-T closed-bore magnet. Fahlbusch showed that for pituitary tumors and low-grade gliomas this system had a major advantage over other systems. A second answer was driven by the Israeli surgeon Moshe Hadani and his group. Initially introduced in 2001 by Hadani and collagues, the PoleStar (Medtronic Navigation, Louisville, Colorado) N-10 iMRI offered an open-configuration, portable 0.12-T magnet that required only modest remodeling of the operative suite. The device was stored in what amounted to a small garage within the operating theater. The N-10’s compact size and low magnetic footprint allowed units to be integrated into multiple institutions’ conventional operating rooms. Despite a slightly increased time for induction of anesthesia and intubation, the units made a significant and positive impact on the safety and completeness of tumor resection in adult and pediatric intracranial procedures. PoleStar recently introduced a higher-field (0.15-T) N-20 ( Fig. 4 ), and initial evaluations confirm that the accuracy, versatility, and quality of this new-generation iMRI scanner are at least as good as the N-10. Clearly further clinical analysis of the accuracy on clinical cases using the N-20 is needed to confirm that these results will bear out in surgical reality.
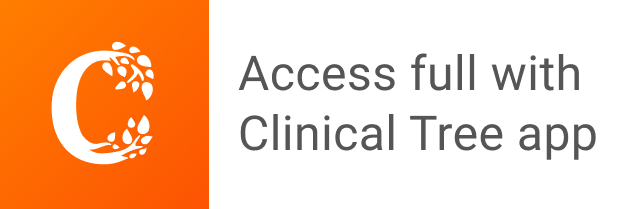