Pediatric Stroke and Congenital Disease
Seena Dehkharghani
Nilesh K. Desai
Neurovascular diseases affect the neonate, infant, and child with a frequency greater than previously recognized and encompass a pathologically heterogeneous gamut of diseases with potentially disastrous consequences for the developing human brain.1,2 The immature brain is unique, both in terms of its normal physiology and its response to injury, and in both respects changes dynamically with age. As in the adult, the childhood neurovascular network represents a highly complex system designed to ensure the steady-state delivery of nutrients and to expel by-products of metabolism, with an elaborate (albeit potentially underdeveloped) autoregulatory system to protect against significant physiologic fluctuations.1,2,3,4,5,6 This reflects the intricate system coupling cerebrovascular perfusion with brain metabolism and central nervous system (CNS) function, as first postulated by Roy and Sherrington7 in the late 19th century, and underlies the principal physiologic parameters intended for interrogation by the techniques presented herein.
Although a large variety of methods have been proposed for the evaluation of cerebral blood flow (CBF) and its related parameters, limitations owing to availability, accuracy, requisite technical expertise, examination duration, invasiveness, and potentially harmful ionizing radiation have substantially diminished the usefulness and clinical applicability of many. Particularly with regard to the latter three considerations, the array of options for perfusion imaging in children is truncated as a consequence of the need for sedation or anesthesia in the uncooperative child and due to ethical concerns over the potentially deleterious effects of ionizing radiation in the pediatric patient. Several such techniques will be mentioned only briefly throughout the course of this chapter for completeness or as a historical treatment; however, the following sections will focus primarily on those applications believed by the authors to best represent the existing options for safe and practical perfusion imaging in this age group.
Background
Cerebrovascular diseases in childhood can be classified as disorders of a primary (e.g., acute vascular occlusion resulting from vascular embolism or arteriovenous malformations) or secondary (suggesting a more systemic underlying disease state such as sickle cell anemia or inflammatory conditions) nature.1,2,8 The latter includes disorders in which the CNS vascular network may not represent the nexus of the pathologic process, but rather a secondarily involved site of disease.
Embolic phenomena in children represent a smaller fraction of neurovascular disease than in adults, and thus in situ thrombosis may be more commonly encountered in pediatric patients.1,9 In either scenario, both standard anatomic imaging and macrovascular angiographic imaging techniques can fail to reveal the presence of tissue at risk of injury, because underlying abnormalities of perfusion and the microvasculature precede the structural changes detected in these disorders by more conventional applications. Perhaps the largest existing body of data examining pediatric brain perfusion imaging was contributed by the work of Ball and Holland,1 who in 2001 shared their experience following more than 1000 pediatric perfusion cases. Their contributions highlighted the benefits of perfusion imaging in offering critical information for clinical decision making, aiding in prognostication and potentially guiding therapy. The preceding may also be influential when considering nonneurovascular disorders, such as brain tumors, offering potentially valuable information in preoperative planning or in following response to therapy (see Chapters 55 through 59). Other potential applications of perfusion imaging in this population include the evaluation of pre- and perinatal CNS injury (perinatal asphyxia, hypoxic ischemic encephalopathy), intoxications and metabolic diseases, head trauma, epilepsy, and the characterization of perfusion changes during maturation of the developing brain.1,2,3,8,10,11,12,13,14,15
Techniques in Pediatric Perfusion Imaging
Many techniques have been developed for the interrogation of CBF and its related parameters, each with a unique profile of advantages and applications; however, inherent limitations have restricted the use of many of these techniques, particularly in the context of pediatric neuroimaging. The earliest efforts to evaluate brain perfusion in childhood employed variations of the nitrous oxide technique proposed originally by Kety and Schmidt16 in the 1940s. Briefly, the technique applies the Fick principle to the uptake of nitrous oxide by the brain; this postulates that the quantity of any substance taken up by an organ is proportional to the total amount of the substance delivered to that organ, less the amount removed by venous drainage during that same period, multiplied by the blood flow to that organ. When carefully applied, the technique may offer an
accurate measure of CBF, as well as other physiologic parameters such as the cerebral metabolic rate of oxygen (CMRO2) and cerebrovascular resistance. The technique, in its initial form, was unsuitable for use in young children due to the need for withdrawal of fairly large quantities of blood and the considerable cooperation required of study subjects. Technical modifications were proposed by Baird and Garfunkel,17 and subsequently by Kennedy and Sokoloff18 in the years that followed, mitigating challenges relating to the volume of required blood and sedation, respectively. Although its validation against existing gold standard techniques such as bubble transfer flowmetry were highly promising, the technique would not find a permanent place in the clinical armamentarium due primarily to its invasive nature and technical challenges.
accurate measure of CBF, as well as other physiologic parameters such as the cerebral metabolic rate of oxygen (CMRO2) and cerebrovascular resistance. The technique, in its initial form, was unsuitable for use in young children due to the need for withdrawal of fairly large quantities of blood and the considerable cooperation required of study subjects. Technical modifications were proposed by Baird and Garfunkel,17 and subsequently by Kennedy and Sokoloff18 in the years that followed, mitigating challenges relating to the volume of required blood and sedation, respectively. Although its validation against existing gold standard techniques such as bubble transfer flowmetry were highly promising, the technique would not find a permanent place in the clinical armamentarium due primarily to its invasive nature and technical challenges.
Although a detailed discussion of the theory behind the nitrous oxide technique is beyond the scope of this chapter, it may be worth noting that this and related techniques are limited in their ability to provide anatomically localized blood flow and may suffer from errors relating to the site of venous blood sampling, contamination of withdrawn venous blood by extracerebral sources, asymmetries in cerebral venous drainage, and errors due to the variability of the partition coefficient of the tracer used among subjects of different ages or in certain pathologic conditions.19
Several less-invasive techniques would gain popularity in the subsequent decades, including gamma-emitting radioactive isotope imaging and positron emission tomography (PET) tracer-based perfusion, which provided the potential for greater anatomic localization and potentially quantitative CBF measurements, but were potentially limited by availability, their often time-consuming nature, and continued concerns over the effects of ionizing radiation in children. Similarly, both stable xenon and dynamic bolus passage of nondiffusible iodinated contrast agents coupled with cine computed tomography have been proposed in children; although potentially valuable in their ability to derive anatomically localized quantitative or semiquantitative measures of CBF, radiation risks have again limited their usefulness in this population, particularly if repeat or serial measurements are necessary.
Lastly, sonographic techniques of varying forms can offer a noninvasive, portable, and innocuous technique for interrogating the cerebral circulation, however, these may be limited by their significant operator dependency, the incomplete panel of perfusion indices derived from their use (generally being limited to parameters such as velocity or CBF volume [CBFV]), and in their limited anatomic localization. Optical spectroscopy techniques (described below) are emerging and show promise in certain settings, however, their use in clinical practice remains limited and further validation is pending.
The advent of magnetic resonance imaging (MRI) sequences, sensitized to microvascular flow with varying forms of perfusion weighting, introduced an entirely new family of techniques for noninvasive or minimally invasive perfusion analysis. These technical developments combined the benefits of exquisite anatomic detail with potentially quantitative and semiquantitative imaging and were free of concerns over ionizing radiation. It is the opinion of the authors that perfusion MRI represents the most practical and clinically relevant technique for perfusion analysis in pediatric clinical practice, and the remainder of this chapter will be discussed in this context.
Perfusion MRI sequences can be easily added to most standard protocols and can be particularly well suited to pediatric imaging, where the small cross-sectional area of the child’s brain and faster heart rate may contribute to image quality. Several fundamentally unique techniques for MRI perfusion exist, and their theoretical and technical aspects are detailed elsewhere throughout this text. Among these, T2*-weighted gadolinium-enhanced dynamic susceptibility contrast (DSC) and arterial spin labeling (ASL) techniques are most commonly used in pediatric neuroimaging. The ASL techniques can be of particular interest in this population, given their noncontrast nature, which makes them a uniquely attractive option when considering imaging of the very young child, or in settings necessitating repeat or serial examinations or imaging after pharmacologic or physiologic intervention (e.g., acetazolamide).1,2,3,8,15,20,21,22 Furthermore, these techniques offer the possibility of quantitative CBF measurements, which can facilitate comparison among populations of patients. Notwithstanding the preceding, ASL techniques have traditionally been used less frequently in many institutions, in part because they can be more time-consuming and often require technical expertise and thus have been historically limited to research applications, although this landscape might be evolving.
ASL techniques now represent a large family of pulse sequences, with the common thread of using magnetically labeled blood water upstream from a region of interest, followed by tissue perfusion imaging using the T1 magnetization of these tagged arterial spins as a freely diffusible tracer following a preset delay. In this manner, pair-wise subtraction of a “labeled” image from a “control” image is performed to generate a difference image, from which absolute CBF (in cubic centimeters per 100 g per minute) can be derived, provided that accurate estimates of several physiologic and imaging variables can obtained. Signal changes from tagged, inflowing spins in the experiment can be in the 2% range, making the technique inherently somewhat signal limited and necessitating repeated averages of the experiment.20,23 The advent of high-field imaging, together with several technical modifications to the original ASL prescription, has proven promising in further mitigating some of these concerns.8,20,23,24,25
Several technical challenges relating to ASL in the neonate and infant should be noted. The lower CBF and longer blood T1 in this age group can engender contamination of “control” images by tagged arterial spins, and
consequently areas of absent signal in computed CBF maps that have been shown to increase in severity at decreasing CBF.3,20,26 Additionally, the subtraction of labeled from control images can introduce considerable errors if motion between the acquisitions cannot be controlled or corrected, and this should be considered when imaging small children.
consequently areas of absent signal in computed CBF maps that have been shown to increase in severity at decreasing CBF.3,20,26 Additionally, the subtraction of labeled from control images can introduce considerable errors if motion between the acquisitions cannot be controlled or corrected, and this should be considered when imaging small children.
Notwithstanding the above, several anatomic and physiologic differences in the developing brain may serve to augment the quality of ASL imaging in children. First, the higher velocities in healthy children established from Doppler ultrasound studies provide for less relaxation of tagged spins proximal to the tissue of interest and can reduce arterial transit artifacts, thus decreasing the confounding effects of this phenomenon on blood flow measurements. Second, the intrinsically higher water content of the childhood brain serves to augment equilibrium magnetization, as well as prolong T1 and T2 relaxation, further contributing to tracer lifetime and signal.
The selection of ASL sequences requires attention to a variety of technical factors, which are detailed elsewhere in this book, and may be largely governed by the options available to an examiner. The authors herein have employed a “pulsed” variety of arterial spin tagging, primarily to avoid concerns over prolonged labeling periods and radiofrequency power deposition common among “continuous” labeling schemes.3,27,28,29,30,31,32,33 The advent of “pseudo-continuous” iterations may provide an ideal labeling scheme in this population, but at the time of this review, they were not commercially available on certain vendor systems.3,34
ASL techniques have been applied in a variety of clinical and research settings, including cerebral perfusion in normal children, following ischemic stroke, in the surveillance of preterm infants, in congenital heart disease, and in the mapping of cortical activation, but they can be informative in a variety of other disease states (Figs. 54.1 and 54.2).15,35,36,37,38,39,40
In contrast to ASL, the DSC techniques employ dynamic bolus passage methodology coupled with a rapid spin or gradient echo-planar imaging (EPI) sequence. Their use in pediatric patients may require efforts to optimize imaging parameters to best suit a specific patient, including attention to the scanner field strength, injection rates, sequence parameters, underlying pathology, and patient age or size (discussed below).24,25,41,42,43 In younger children, efforts should be made to reduce slice thickness and field of view, which when coupled with signal-to-noise ratio (SNR) improvements predicted at higher field strengths can be advantageous when available and prudent. Susceptibility artifacts commonly compromising imaging quality in gradient refocused EPI near the air–tissue interface of the frontal sinuses and along the central skull base may prove less problematic in young children, in whom air cell development may be absent or incomplete.1,8 Anatomic imaging is always advised in conjunction with perfusion imaging of any variety; standard imaging sequences are frequently modified for pediatric imaging, and although an exhaustive discussion of the specific considerations in childhood MRI is beyond the scope of this chapter, the authors refer the reader to several excellent resources and reviews on this topic.24,25,41,42
As in the adult, processed DSC perfusion maps are interpreted within the context of the specific clinical question. In comparison with the ASL techniques, signal changes with DSC imaging can be in the range of 15% to 30% or more from baseline, depending on scan parameters and contrast dosage.8 The first pass of contrast in dynamic bolus passage has been reported in the 10- to 13-second range and noted to occur slightly faster than generally observed in adults.11 As in the adult, validity of the DSC technique presupposes the presence of an intact blood–brain barrier (BBB) to satisfy the isolation of the tracer to the intravascular compartment. Past studies have suggested that the integrity of the BBB is satisfactory, even in young children, making DSC perfusion imaging a viable option in this age group. Normal relative blood volume values parallel those encountered in the adult, with normal gray matter to white matter ratios between 3 to 4 in 1 for children, although greater ratios may be demonstrated in the very young (<2 to 3 years old).1 CBF, bolus arrival time, and transit time parameters should be equal between hemispheres in the normal brain; a potential caveat with regard to the preceding would be the possibility of encountering an apparent delay in bolus arrival time or time to peak within an occipital lobe supplied by the posterior circulation in the setting of a contralateral “fetal” posterior cerebral artery arising from the anterior circulation. The middle cerebral artery territory is often noted to enhance and subsequently washout earliest and can demonstrate the highest flow among the cardinal vascular territories of the circle of Willis.1
A third MR perfusion technique uses phase-sensitive imaging to collect signal changes reflecting perfusion-induced intravoxel incoherent motion. Although phase contrast techniques have been recently revisited in pediatric perfusion imaging, they are not commonly employed for these purposes and are presented here primarily for completeness.44
Special Consideration in Pediatric Neuroimaging
Imaging the pediatric patient, by whatever modality, does not seamlessly translate from adult imaging methodology nor does imaging within pediatrics itself translate seamlessly, for instance, from the neonate or infant to the older child. Pediatric imaging requires a subset of honed skills and considerations by all those involved, including technicians, sedating physicians, radiologists, and physicists. MRI for all children, especially the preterm, term neonate, and infant, poses notable potential risks, just as in adults. One especially important aspect in children involves the potential risk of hyperthermia and hypothermia. The necessarily cool ambient temperature of the MRI suite, radiofrequency deposition during the examination, wrapping or swaddling of the youngest of patients, and even seemingly minor issues such as the presence of a small cooling fan in the bore of the magnet and time in the suite without active scanning seriously complicate the potential end-result temperature effects for a child. This is even further compounded by the often tenuous nature imposed upon children by various acute or chronic diseases and the need for many children to be sedated for MRI studies, the latter of which independently impairs thermoregulatory control.45,46 Multiple studies have evaluated the temperature effects of MRI with and without sedation. In a study of American Society of Anesthesiologists class I and II patients, ranging in age from 1.4 to 4.4 years, sedated primarily with propofol, significant increases in body temperature up to 0.9°C at 1.5T and up to 1.0°C at 3.0T were noted.47 Bryan et al.48 demonstrated similar results, with the majority of a group of subjects ranging in age from 2 to 33 months sedated with chloral hydrate, with 80% demonstrating an increase in tympanic temperatures up to 1.3°C, but with 14% of patients experiencing a decrease in temperature of up to 0.3°C. One of the largest and most exhaustive studies addressing this issue, occurring partly at the authors’ own institution, evaluated 374 children sedated primarily with propofol and nonsedated children of various ages with different protocols and magnetic strengths (1.5T and 3.0T). Twenty-two patients demonstrated a change of temperature before and after MRI of greater than 1°C. Temperature changes among all patients differed significantly between sedated (more likely to incur a decrease in temperature) and nonsedated patients, and
also between 1.5T and 3T (with a greater likelihood for increased temperature at 3.0T compared with 1.5T). In addition, younger patients were more likely to incur larger decreases in temperature than older, nonsedated counterparts, among whom temperature increases were more likely.49 Based on the current literature, although continuous monitoring of patient temperature is not clearly beneficial, at the authors’ institution, blankets and even warmed gel packs are generally used for our youngest and sedated patients and the in-bore fan is turned to the lowest setting. In the older, nonsedated population, patients are scanned without such warming measures and instead regular communication with such patients during the course of the examination suffices to avert dangerous hyperthermia. As a further precaution, the authors do not routinely admit patients to MRI with uncontrolled core temperatures greater than 38°C.
also between 1.5T and 3T (with a greater likelihood for increased temperature at 3.0T compared with 1.5T). In addition, younger patients were more likely to incur larger decreases in temperature than older, nonsedated counterparts, among whom temperature increases were more likely.49 Based on the current literature, although continuous monitoring of patient temperature is not clearly beneficial, at the authors’ institution, blankets and even warmed gel packs are generally used for our youngest and sedated patients and the in-bore fan is turned to the lowest setting. In the older, nonsedated population, patients are scanned without such warming measures and instead regular communication with such patients during the course of the examination suffices to avert dangerous hyperthermia. As a further precaution, the authors do not routinely admit patients to MRI with uncontrolled core temperatures greater than 38°C.
As noted previously, because preterm and term neonatal imaging is especially challenging, requiring specifically trained staff, patient preparation, transportation, and use of extensive MR compatible equipment (e.g., respirators, pumps and lines), a streamlined, standardized approach is ideal. One promising advance for imaging of the neonate is the use of MR-compatible incubator systems, which afford such patients a well-controlled microenvironment that minimizes exposure of the often tenuous neonate to potential environmental and logistical harms of undergoing an MRI examination, yet still being intimately monitored (Fig. 54.3).50 Although the use of such MR compatible incubators is the standard of care at many tertiary neonatal intensive care centers, limited patient volume at smaller institutions and cost currently prohibit widespread use.
Regardless of age, standard high field strengths (1.5T and 3.0T) are employed in all aspects of pediatric imaging. Inherent challenges of 3.0T exist for children as in adults, including field inhomogeneity and artifact elaboration as well as undesired issues of longer T1 relaxation times. The latter principally complicates imaging of the neonatal brain due to its higher water content compared with the adult brain, thereby yielding fairly poor gray–white matter differentiation. Such challenges are satisfactorily overcome with the use of longer repetition times (TR), and the use of inversion recovery and magnetization prepared three-dimensional imaging (e.g., magnetization-prepared rapid gradient echo). Neuroimaging at 3.0T benefits children in the same way as in adult imaging with improved SNR and the potential for higher spatial resolution and shorter scan time.
As in adults, modern MRI of the pediatric brain primarily used multichannel phased array coils due to higher SNR, improved spatial resolution, and the efficiencies of parallel imaging afforded by such coils compared with transmit receive quadrature design coils. Despite potential concerns for radiofrequency deposition, given the use of a magnet’s inherent body coil for radiofrequency pulse transmission (with the use of receive only phased array coils), specific absorption rate limits are not typically reached for routine imaging, even in neonates. It is worth mentioning, however, that even in large tertiary pediatric centers, dedicated pediatric-phased array coils might not be available thru some MRI vendors and, therefore, it is not uncommon for either a phased array knee coil or an adult head coil to be used. Although use of these latter devices is certainly adequate and again representative of many pediatric centers, the multifaceted improvement in SNR, spatial resolution, and optimal realization of efficiencies afforded by parallel imaging for coils that intimately conform to the various sizes of the pediatric head is clear.51
Peripheral intravenous lines inserted for children vary with age, typically with 22- to 24-gauge angiocatheters used for the smallest of children, whereas older children are commonly able to accommodate intravenous lines of 18 to 20 gauge. The maximal flow rate for 24-, 22-, and 18- to 20-gauge catheters are 1.5, 3, and 5 cc/s, respectively. At the authors’ institution, because routinely inserted angiocatheters for radiologic procedures are most always 22 gauge maximally, and because of anecdotal concerns over patient discomfort at high bolus rates, flow rates for power injected gadolinium perfusion imaging are capped at 2 cc/s (standard gadolinium dosing at 0.2 cc/kg or 0.1 mmol/kg). No preferential side or site (dorsal hand or ventral forearm or antecubital fossa) is mandated at the authors’ institution in MRI and anecdotally no adverse effects such as extravasation have occurred as a result of where the intravenous line is placed. This is in agreement with other reports that affirm this same opinion.52,53 Gadolinium-enhanced perfusion imaging with power injection is, however, admittedly difficult in neonates, infants, and toddlers due to smaller
angiocatheters and limited doses, and thus the use of power injectors is largely limited to older children. In addition, power injection carries with it a higher risk of extravasation.8 Hand injection is, therefore, most commonly used in this young pediatric population. Laswad et al.54 recently described an easily reproducible method of gadolinium bolusing for DSC perfusion-weighted imaging (PWI) producing sharp declines and inclines in signal intensity on concentration time curves. In this method, gadolinium is carefully loaded onto the front end of a saline bolus within extension tubing, thereby limiting mixing of the gadolinium with the saline. This creates a single administration bolus negating the need for switching syringes between gadolinium and saline flush bolus and allowing saline to by default chase the front-loaded gadolinium.
angiocatheters and limited doses, and thus the use of power injectors is largely limited to older children. In addition, power injection carries with it a higher risk of extravasation.8 Hand injection is, therefore, most commonly used in this young pediatric population. Laswad et al.54 recently described an easily reproducible method of gadolinium bolusing for DSC perfusion-weighted imaging (PWI) producing sharp declines and inclines in signal intensity on concentration time curves. In this method, gadolinium is carefully loaded onto the front end of a saline bolus within extension tubing, thereby limiting mixing of the gadolinium with the saline. This creates a single administration bolus negating the need for switching syringes between gadolinium and saline flush bolus and allowing saline to by default chase the front-loaded gadolinium.
Sedation is a necessary part of many modalities within pediatric imaging, especially within MRI. Although sedation may not be necessary for the majority of neonates once swaddled and fed, sedation is necessary for essentially all children until late in the first decade of life with few exceptions. Sedation remains commonplace for many patients with acute or chronic diseases or neurodevelopmental deficiencies, however, independent of age.
A pertinent topic to address is the potential effect of sedation on cerebral perfusion dynamics, with this brief discussion largely limited to the effects of propofol, given its widespread usage in both moderate sedation and the induction and maintenance of general anesthesia. In a small group of normal subjects studied by Biagi et al.,15 15 pediatric patients, including six awake, six sedated with halogen, and six sedated with propofol, were evaluated by ASL PWI. In this group of patients, mean CBF values in gray matter was approximately 9% higher in patients sedated with halogen compared with awake children, whereas CBF did not significantly differ between awake children and those sedated with propofol. In contradistinction, Veselis et al.55 demonstrated that with sedative and hypnotic doses of either propofol or thiopental in 10 adult patients, global regional CBF, as assessed by O15-labeled water PET, decreased by approximately 15% and 27%, respectively.15 This correlates well with several other studies evaluating the effect of propofol on regional CBF also using O15-labeled water PET. Using this technique, Fiset et al.56 evaluated absolute and normalized regional CBF in five healthy adults at multiple concentrations of propofol. At concentrations where the patient is unconscious and unresponsive but spontaneously breathing, regional CBF decreased globally by 20.2%. Normalized regional CBF decreased in the thalamus and parieto-occipital cortices, including the cuneus, precuneus and posterior cingulate, and orbitofrontal cortices, with variations in blood flow to the thalamus statistically correlating with flow in the reticular formation of the midbrain. Conversely, regional CBF correlated positively with the propofol dose in other areas, including the cerebellum, medial frontal cortex, and left temporal pole. A similar study also using O15-labeled water PET demonstrated a decrease in regional CBF of 46% to 55%, with the most significant decrease in the thalamus, parietal lobe, and precuneus region. No areas of increased regional CBF were encountered in this group.57 These latter studies therefore corroborate the preferential activity of propofol on the reticulothalamic system and certainly support propofol causing globally reduced regional CBF. Nevertheless, these studies remain few in number with few subjects, as study design necessarily relies on healthy adult volunteers, and understandably such studies have therefore not been replicated in children. Furthermore, even if depression of regional CBF may be assumed to occur similarly in children, despite results by Biagi et al.15 to the contrary, further studies are clearly needed to understand how and where exactly such sedative agents affect the developing brain. This understanding is crucial, as the untoward introduction of an incompletely appreciated bias could confuse and void comparative analysis of perfusion imaging within and between sedated and nonsedated children. Similarly, anesthetic-induced changes in CBF may not translate equally to changes in cerebral blood volume (CBV), and, therefore, induced changes in CBF may not, in and of themselves, completely describe the effect such agents have on perfusion dynamics.58
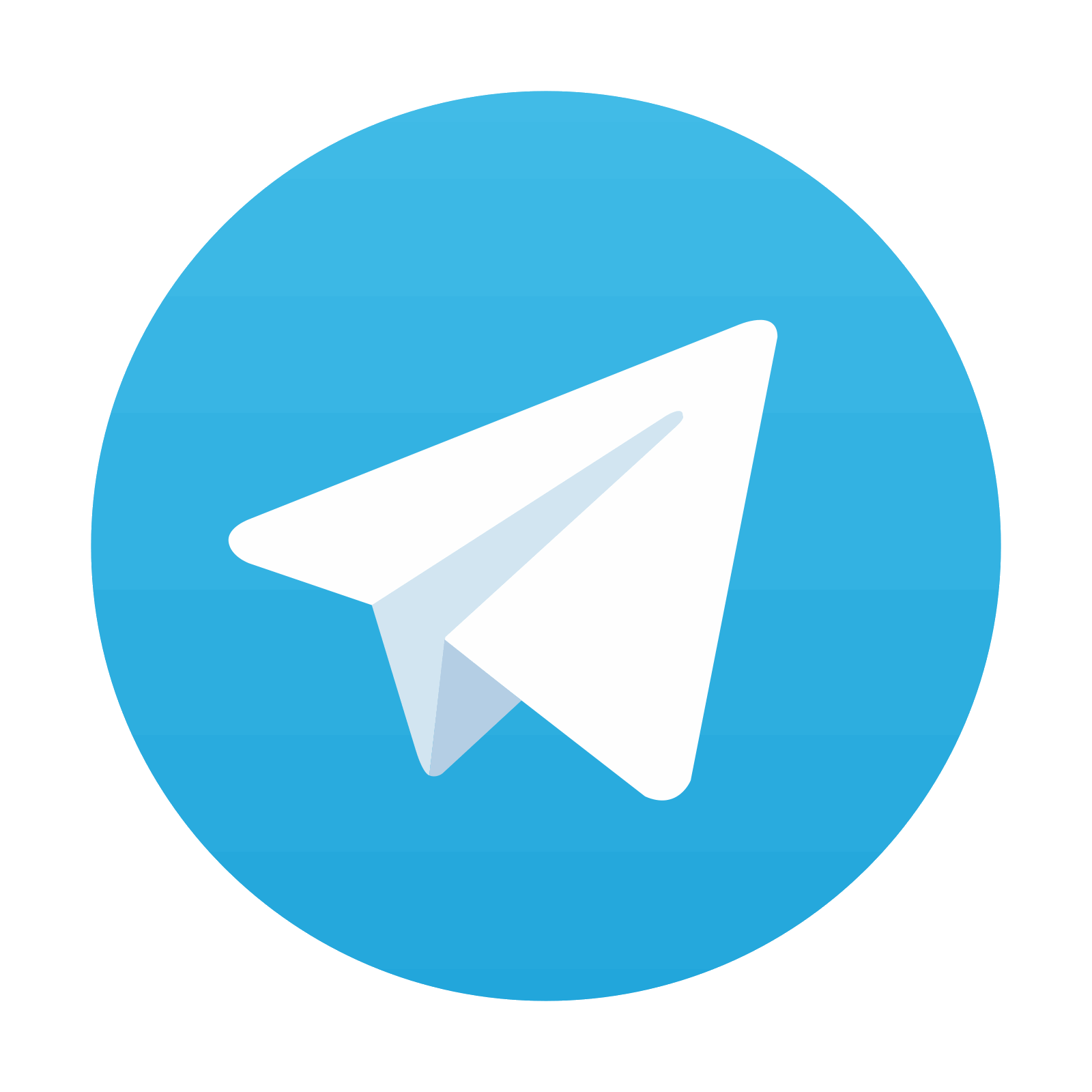
Stay updated, free articles. Join our Telegram channel
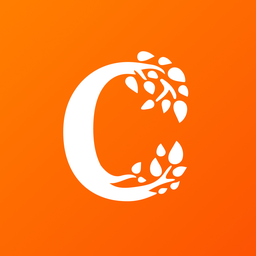
Full access? Get Clinical Tree
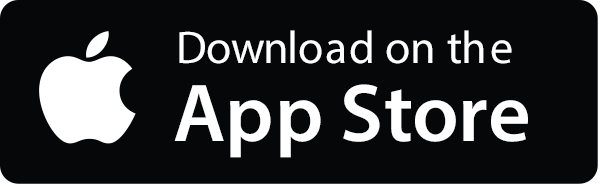
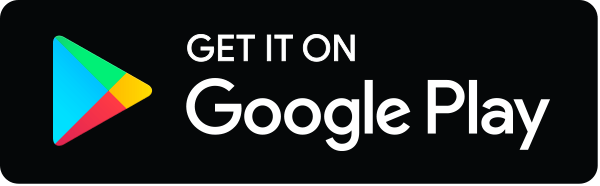