Allogeneic
Autologous (adult stem cells)
Embryonic stem cells
Induced pluripotent stem cells (iPSCs)
Fetal cardiomyocytes
Adipose-derived stem cells
Human umbilical cord-derived cells
Resident cardiac stem cells (cardiospheres)
Human allogeneic mesenchymal stem cells
Epicardium-derived stem cells
Skeletal myoblasts
Mesenchymal stem cells (MSCs)
Bone marrow derived:
Endothelial progenitor cells (EPCs)
Mononuclear fraction
CD34+ fraction
Embryonic Stem Cells
Embryonic stem cells (ESCs) have the broadest developmental potential (pluripotent) since they can give rise to cells of all three embryonic germ layers. Functionally intact cardiomyocytes have been generated from human ESCs in vitro [10], and in a mouse model ESC-derived cardiomyocytes, when injection into the infarcted myocardium, formed stable grafts and subsequently contracted in synchrony with adjacent cells [11].
However, the use of ESCs is associated with teratoma formation in animal models [12] which raises concerns regarding their malignant potential. This, together with the ethical and legal issues surrounding the use of human ESCs, has hampered further research efforts, and current focus is on other sources of stem cells for clinical application of cardiac repair.
Unlike ESCs, autologous adult stem cells appear virtually free from the risks of teratoma formation and immune rejection although display more limited differentiation potential. Adult stem cells that have been studied to date include induced pluripotent stem cells, adipose-derived stem cells, tissue-resident cardiac stem cells, skeletal myoblasts, mesenchymal stem cells, circulating endothelial progenitor cells, and most commonly bone marrow-derived mononuclear stem cells.
Induced Pluripotent Stem Cells
An exciting alternative to ESCs is emerging in the form of inducible pluripotent stem cells (iPSCs) which are adult somatic cells that have been successfully reprogrammed back to an undifferentiated pluripotent state by inserting four genes, Oct3/4, Sox2, KL4, and c-Myc, into differentiated somatic cells [13–15]. These cells have the morphological phenotype of ES cells and have been demonstrated in vivo and in vitro to have the same differentiation potential as ES cells (able to form all three germ layers). Functioning cardiomyocytes have already been produced from iPSCs [16] demonstrating their potential use in cardiovascular regenerative medicine although there remain theoretical concerns regarding tumor genesis.
Adipose-Derived Stem Cells
Adipose tissue has been investigated as a source of adult progenitor/stem cells for the purposes of cardiac repair as this tissue contains a heterogeneous mixture of endothelial, hematopoietic, and mesenchymal stem cells which can be easily harvested by liposuction [17]. Preclinical studies have shown that adipose-derived stem cells (ADSCs) are associated with improvement in ejection fraction in animal models of myocardial infarction, and neoangiogenesis via paracrine factors has been postulated as a potential mechanism of action of ADSCs [18, 19]. Clinical trials are currently ongoing and include the APOLLO study (ClinicalTrials.gov Identifier: NCT00442806) and PRECISE study (ClinicalTrials.gov Identifier: NCT00426868), which are assessing the safety and efficacy of ADSCs in acute myocardial infarction and chronic myocardial ischemia, respectively.
Resident Cardiac Stem Cells
The heart has been considered a terminally differentiated organ lacking self-renewal capabilities, but this dogma has been challenged recently, particularly with the demonstration of continued cell division within the adult heart following injury such as myocardial infarction [1]. Several independent investigators have now isolated and identified resident cardiac stem cells which are capable of differentiating into multiple cardiac cell lineages such as cardiomyocytes and vascular smooth muscle cells [20, 21]. These cells have been studied in animal models of myocardial infarction with beneficial outcomes in terms of reducing infarct size and improving LV function [22]. Resident cardiac stem cells are clearly an attractive option for cardiac repair although a harvesting technique remains to be perfected and clinical trials of safety and efficacy are still awaited. The ongoing CArdiosphere-Derived aUtologous Stem CElls to Reverse ventricUlar dySfunction (CADUCEUS) study, which is a phase 1 study recruiting 30 patients to receive autologous cardiosphere-derived stem cells, will hopefully answer some of these questions (ClinicalTrials.gov Identifier: NCT00893360).
Epicardium-Derived Progenitor Cells
As mentioned previously the zebra fish can fully regenerate its heart after injuring up to 20 % of the left ventricle [23]. Recent experimental evidence suggests that this regeneration may occur through limited dedifferentiation of existing cardiomyocytes followed by proliferation [24] and through activation and expansion of surrounding epicardial tissue which supports the regenerating myocardium [25]. These studies in zebra fish suggest that the epicardium may play an important role not only in adult heart repair but also during continuous growth of the adult heart [26]. A subset of epicardium-derived cells (EPDCs) expressing known markers of stem cells, c-kit and the CD34, has been identified in the subepicardial space of fetal and adult human hearts [27]. Experimental studies have demonstrated that EPDCs have the potential to differentiate into cardiomyocytes [28] and intramyocardial injection of human EPDCs in a mouse model of myocardial infarction has been shown to improve cardiac function supporting the hypothesis that EPDCs may play an important role in cardiac repair.
Skeletal Myoblasts
Skeletal myoblasts have been widely studied due to several favorable characteristics: (a) their muscle phenotype with hope for contractile function when transplanted into the heart, (b) autologous source of cells avoiding immune rejection, (c) minimal risk of tumor formation after transplantation, (d) and easily harvested, (e) cell numbers can be increased significantly prior to transplantation, and (f) relatively resistant to ischemia which may help long-term survival in the hostile environment within the diseased heart. Early preclinical animal studies have demonstrated the ability for skeletal myoblasts to engraft, form myotubules, and enhance cardiac function after transplantation into infarcted myocardium [29]. However, not all of the preclinical studies have provided positive results. For example, consistently, studies have demonstrated significant cell loss: up to 84 % loss in the first 24 h and death of the transplanted myoblasts within a few days [30, 31]. Furthermore, transplanted cells downregulate the major adhesion and gap junction proteins, N-cadherin and connexin43, of the intercalated disc [32] and are functionally isolated from the host myocardium with no evidence of electromechanical coupling [33]. This may account for the increased incidence of arrhythmias seen in hearts after myoblast transplantation [34]. Another limitation is that they remain committed to the skeletal muscle lineage, and hence interest in the clinical application of these cells is decreasing.
Bone Marrow-Derived Stem/Progenitor Cells
The most widely studied of the adult stem cells has been bone marrow-derived mononuclear/stem cells (BMSCs). Bone marrow-derived cells consist of hematopoietic stem cells (HSCs), mesenchymal stem cells (MSCs), and endothelial progenitor cells (EPCs), all of which have been shown in preclinical studies to have the potential to transdifferentiate to a cardiomyocyte phenotype [35–37]. BMSCs can also be characterized by specific cell surface markers, for example, CD34, which is expressed on primitive stem cells and differentiated progenitor cells and is absent on mature hematopoietic cells. Additionally, cell populations can be further distinguished by the lineage marker (Lin), which if present represents cells committed to a particular lineage, and the stem cell factor receptor c-kit and the stem cell antigen-1 (sca-1), which are both markers of primitive stem cells.
BMSCs have been demonstrated to transdifferentiate into multiple unexpected cell types including neural cells [38], skeletal muscle [4], and hepatic cells [39]. This “plasticity” of BMSCs has led to considerable excitement in their use in cell-based therapies and as vectors to deliver therapeutic agents. This is particularly attractive clinically because bone marrow can be readily obtained from patients by direct aspiration or by indirect peripheral harvest following mobilization with cytokines such as granulocyte colony-stimulating factor (G-CSF). Also, if a patient’s own stem cells were taken for ex vivo expansion and directed to differentiate into a cardiac phenotype, immunogenicity issues are unlikely to occur.
In a landmark animal study, Lin−c-kit+ (markers of undifferentiated stem cells) BMSCs were injected directly into the contracting wall bordering a myocardial infarct which had been acutely induced by coronary artery ligation [6]. The transplanted cells appeared to undergo transdifferentiation to cardiomyocytes with newly formed myocardium occupying a significant proportion of the infarcted area with significant improvement in LV ejection fraction just 9 days after cell transplantation. This study at least in part has prompted the rapid translation into clinical trials assessing the safety and efficacy of BMSCs following AMI in humans – these trials are discussed in detail later.
In summary, multiple cell types have been investigated for their potential to be used in cardiac repair. The ideal cell type(s) remains to be identified. However, recent identification and isolation of resident cardiac stem cells offers hope for cardiac-specific stem cell transplantation. Furthermore the cell type to be used may depend on the clinical condition and myocardial substrate being treated. For instance, the angiogenic potential of BMSCs may be most relevant to the AMI setting, while the myogenic potential of skeletal myoblasts may be more applicable to repair of chronically infarcted myocardium. There is ongoing effort to realize the potential of cellular therapy, and scientists together with clinicians continue to work closely to translate the promising preclinical work into meaningful benefits to patients.
Methods of Cell Delivery
There are currently five distinct methods for delivering cell therapy: (1) peripheral infusion, (2) cell mobilization with cytokines such as G-CSF, (3) transvenous via the coronary sinus, (4) intracoronary infusion, and (5) intramyocardial injection – which can be either transepicardial, transendocardial, or trans-coronary-venous injections (Fig. 14.1). All the techniques remain in the early stages of clinical investigation, and it remains to be determined which of these will prove to be the most efficacious approach. A limitation common to all current techniques is the low target area cell retention achieved which has been highlighted in a preclinical study [41] that demonstrated maximal cell retention rates within the myocardium of 11 % with intramyocardial injection, 3 % with intracoronary injection, and 3 % with intravenous infusion. There is ongoing work trying to elucidate the reasons for these losses and finding solutions to improve the efficiency of acute cell retention such as combining the cells with a biological scaffold [42]. Numerous scaffold designs have been created for use in cardiac applications using gelatin, fibrin, collagen, and alginates in the form of gels or 3-dimensional (3-D) scaffolds [43, 44].
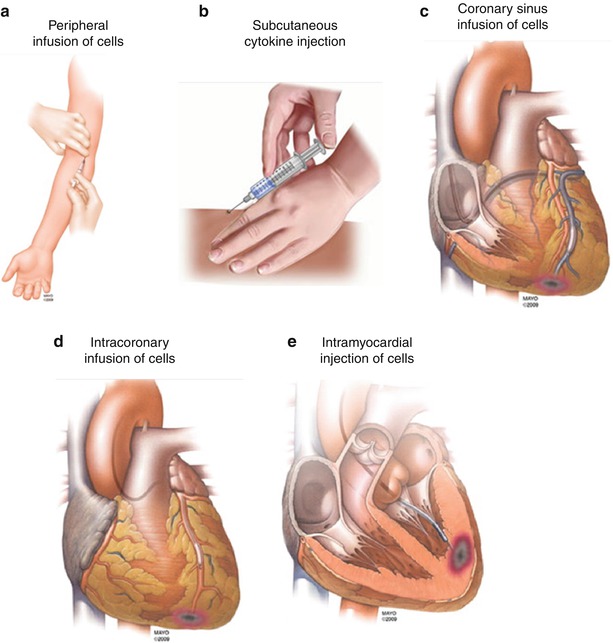
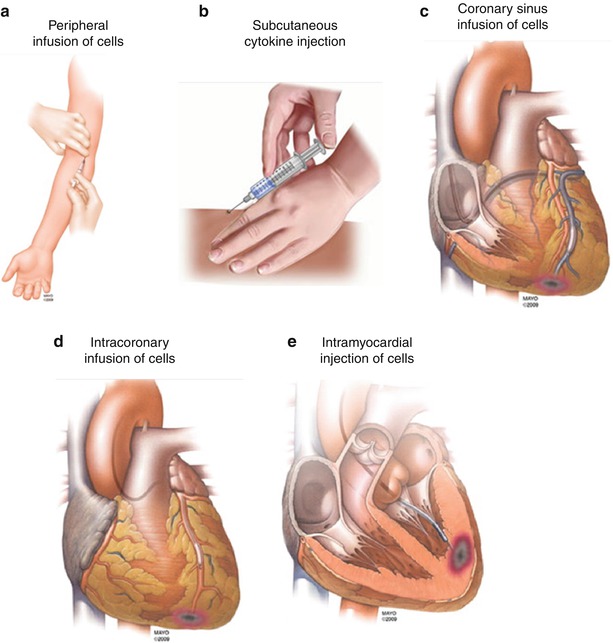
Fig. 14.1
Available methods of delivery for cell therapy. There are currently five different methods for delivering cell therapy: (a) peripheral infusion, (b) cell mobilization with cytokines such as G-SCF, (c) transvenous via the coronary sinus, (d) intracoronary infusion, and (e) intramyocardial injection – which can be either transepicardial, transendocardial, or trans-coronary-venous injections. Adapted from Gersh et al. [40]
Peripheral Infusion
Peripheral intravenous infusion of stem cells is an attractive noninvasive method of stem cell delivery due to its simplicity and applicability. Human bone marrow cells have been shown to “home” to peri-infarct areas when infused into the peripheral circulation of a mouse model of acute myocardial infarct [45]. However, this technique may not be suitable in the chronic heart failure setting, which lacks the temporarily upregulated biological homing signals present in the AMI setting. Another significant limitation is that only a few cells appear to reach the affected area due to trapping of the cells in the microvasculature of the lungs, liver, and lymphoid tissues [46]. Peripheral infusion has been extensively used in animal models, but there has been only one human study to date – a placebo-controlled study assessing primarily the safety of peripheral infusion of allogeneic human mesenchymal stem cells (Prochymal™) after acute myocardial infarction [47]. This was confirmed as a safe delivery method, and the treatment group showed significant improvement in symptoms, LV ejection fraction, as well as evidence of reverse remodelling on cardiac MRI. However, given the already small numbers of injected cells that are known to be retained by the heart, it is likely that more direct interventional injection will be more efficacious.
Cell Mobilization
Cell mobilization provides an indirect method of introducing cell therapy to the injured myocardium. In the clinical setting, one of the most studied mobilizing factors is granulocyte-stimulating factor (G-CSF). Endogenous G-CSF is a potent hematopoietic cytokine that affects progenitor cell proliferation, maturation, and functional activation and enhances the mobilization and recruitment of stem cells from the bone marrow to the blood circulation [48]. G-CSF is also proposed to have a range of direct effects on the myocardium, including creating a favorable environment for homing and engraftment of stem cells, inhibiting apoptosis, and promoting cardiomyocyte survival, including angiogenesis and reducing fibrosis associated with adverse cardiac remodelling [49]. G-CSF is generally well tolerated with most common side effects being bone pain and fevers.
Initial preclinical animal studies [50] and phase 1 clinical trials after myocardial infarction showed that G-CSF treatment appeared to be safe with possible beneficial effects on left ventricular systolic function [51, 52]. However, a subsequent randomized placebo-controlled study of subcutaneous G-CSF after primary PCI for AMI showed no additional improvements in left ventricular function when compared to placebo [53]. This was confirmed in a meta-analysis on the effect of G-CSF in AMI which showed no overall benefit although subset analysis showed there may be benefit limited to patients with LV systolic dysfunction and if the infusion is started early [54]. There has also been concerns raised regarding the potential of G-CSF to cause in-stent restenosis (ISR) as it increases the level of circulating neutrophils [55] which may accelerate the process of neointimal proliferation. One of the initial clinical trials reported an unexpectedly high rate of ISR in ten patients treated with G-CSF following an AM [56]. However, a more recent trial involving patients with large infarcts and late revascularization did not show any increased incidence of ISR [57]. Furthermore, an intracoronary intravascular ultrasound (IVUS)-based study, 5 months after stent insertion for AMI, showed no increase in in-stent neointimal hyperplasia in the G-CSF treatment group compared to placebo [58]. Two small, nonrandomized, studies have shown possible beneficial effects of G-CSF in chronic ischemic heart failure with regard to patient symptoms and improvement in LV function but also raised concerns regarding worsening of angina during the treatment phase [59, 60]. It remains to be determined if G-CSF treatment could be an effective part of a treatment strategy combining several cytokines and/or local stem cell delivery, and there are ongoing trials of its use (e.g., REGENERATE-IHD [61]) which will provide further data regarding this.
Coronary Sinus Infusion
Retrograde infusion of stem cells through the coronary sinus and coronary venous system has been achieved in experimental models which suggest that this method may provide a more uniform delivery of cells with improved cell retention rates [31]. There have been sporadic case reports [62] demonstrating safety of this technique, but there have not been any published clinical trials of this technique. In this procedure, the coronary sinus is cannulated using a guidewire, and an angioplasty balloon is advanced to the selected cardiac vein related to the coronary artery territory to be treated. The venous blood flow is then halted, by prolonged balloon inflation for approximately 15 min, while the stem cells are infused under pressure. There is yet to be any clinical efficacy data regarding this technique, and the main limitations to this approach are the technical difficulties related to the more variable coronary venous anatomy, which can at times be difficult to negotiate with guidewires and balloons. There are also concerns relating to the potential myocardial injury caused by high-pressure retrograde infusion. This technique may not be possible in patients who already have a coronary sinus device in situ such as a LV lead for resynchronization therapy or a coronary sinus annuloplasty device although cells could be administered as an adjunct to the implantation of such devices.
Intracoronary Infusion
Intracoronary infusion is the method that has been most widely adapted in clinical trials of cell therapy, especially after primary angioplasty for AMI. This method has also been used in patients with heart failure secondary to ischemic heart disease, although in this setting occluded coronary arteries may prevent targeted delivery to the infarcted area. The technique employs the skills of balloon angioplasty and therefore can be performed by an interventional cardiologist with minimal additional training. Cells are infused through the central lumen of an over-the-wire (OTW) balloon catheter during low-pressure balloon inflation for 2–4 min (“stop-flow” technique). Standard short (6–10 mm) balloons are used to minimize endothelial injury, and balloons are also undersized by 0.5 mm less than the reference vessel diameter. Balloon inflation time is empirically chosen, and the purpose is to prolong the contact time between the infused cells and endothelium of the coronary macro-/microcirculation as well as to provide an ischemic stimulus which may improve homing of cells to the target myocardium. Inflation time may need to be curtailed in patients who do not tolerate prolonged occlusion of a clinically important coronary artery. In the AMI setting infusion of cells is limited to the infarct-related artery, while in patients with chronic heart failure, cell infusion may be distributed amongst all patent coronary artery and graft conduits. Some more recent animal work suggests that single bolus infusion may be as effective [63] although stop flow seems to be the prominent method in the published trials.
A preclinical study in dogs raised concerns regarding the possibility of microinfarcts caused by intracoronary injection of mesenchymal stromal cells [64], and there is likely to be a safety threshold with regard to the size and dose of cells delivered using the intracoronary route. Reassuringly, the relative safety of this technique has been confirmed in multiple clinical trials, and a recent meta-analysis of 811 patients confirmed that the relative risks of mortality and morbidity, measured by incidence of reinfarction, arrhythmias, restenosis, hospital readmission, and target vessel revascularization, were not significantly increased in participants who received intracoronary BMSCs following AMI compared with controls [65].
Intramyocardial Injection
Intramyocardial injection is the most direct method of administering cell therapy, and there are several advantages of this technique in patients with heart failure and angina. In contrast to the AMI setting, patients with chronic ischemic cardiomyopathy are unlikely to release signals from damaged myocardium to induce stem cell homing, and theoretically it may be more effective to use intramyocardial injection to deliver the cells to the target area. Also, in the chronic setting the coronary artery subtending the infarct/ischemic area may be occluded without significant collateral formation, and thus intramyocardial delivery provides a means for targeting these areas. Furthermore, experimental data using radionucleotide-labelled BMSCs suggest that target area cell retention may be higher with intramyocardial injection when compared to the intracoronary delivery method [66]. Experience of the intramyocardial route has been mainly limited to chronic conditions due to concerns of myocardial perforation and arrhythmia in the acute setting.
There are currently three methods for intramyocardial injection of cells: open surgical transepicardial injection, percutaneous transendocardial injection, and trans-coronary-venous intramyocardial injection of cells. Earlier clinical trials have relied on surgical transepicardial injection of cells where results are confounded by simultaneous coronary bypass surgery [34, 67–69]. When bypass is not indicated, percutaneous delivery may provide comparable efficacy with reduced risk. Studies in swine models of chronic ischemic heart failure comparing percutaneous and surgical transplantation of skeletal myoblasts have demonstrated that both approaches lead to similar improvements in LVEF and reverse remodelling [70].
Percutaneous transendocardial injection is usually performed via 8Fr femoral access although brachial access for transendocardial injection of stem cells has been reported [71]. A percutaneous approach allows this therapy to be delivered to the higher-risk population and also provides an option for repeated therapy if required. The main additional risks of this approach are ventricular perforation leading to pericardial effusion/tamponade and procedure-related ventricular arrhythmias although the reported incidences of these have been low in the published clinical trials. A deflectable tip injection needle catheter is advanced retrogradely across the aortic valve, and cells can be injected directly into any area of the left ventricular endocardium. There are four commercially available catheter systems currently being used for transendocardial cell delivery: the MyoStarTM (Biosense Webster), the MyoCath™ (BioHeart), the HelixTM (BioCardia), and the StilettoTM (Boston Scientific) catheter delivery systems (Fig. 14.2). The MyoStar™ catheter system utilizes a 3-dimensional (3-D) navigation system to guide injection, and the other three catheters are guided fluoroscopically therefore providing 2-dimensional orientation only. The first two catheter systems are introduced without a guidewire so require manipulation when advanced from the femoral artery and across the aortic valve while the second two catheters are introduced over a traditional guidewire system.
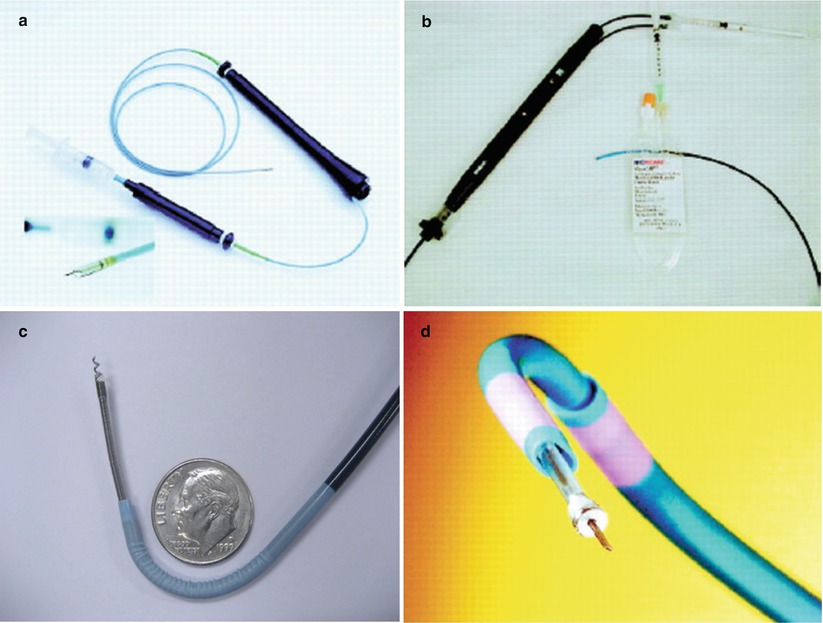
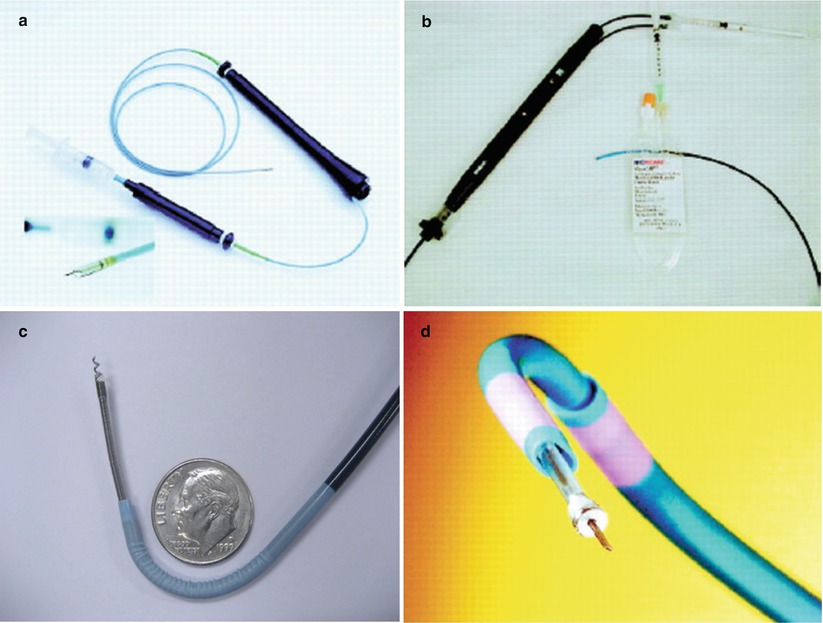
Fig. 14.2
Four commercially available catheters, which are currently under investigational use in clinical trials: (a) MyoStarTM (Biosense-Webstar), (b) MyocathTM (BioHeart), (c) HelixTM (BioCardia), (d) StilettoTM (Boston Scientific)
The MyoStarTM mapping catheter system utilizes nonfluoroscopic guidance using the NOGA® (Biosense Webster) magnetic electromechanical system for intramyocardial navigation and mapping (Fig. 14.3). This technique uses ultralow-intensity magnetic fields generated by a triangular magnetic pad positioned beneath the patient and location sensor on the tip of the mapping catheter, which helps determine the real-time location and orientation of the catheter tip inside the left ventricle. The NOGA® system analyzes the movement of the catheter tip at the location of an endocardial point, timed with systole and diastole, and compares it with the movement of neighboring points – the percentage difference represents the degree of mechanical function at that endocardial point. The mapping catheter tip is also capable of measuring endocardial voltage potentials and an electrical map is constructed concurrently with the mechanical map. Healthy endocardium delivers a resting unipolar potential of 15 mV, whereas voltage values less than 6.9 mV reflect scar tissue; potentials between 7 and 15 mV suggest viable myocardium [72]. When the map is complete, all the data points are integrated by the NOGA® workstation and represented in a 3-D color-coded reconstruction of the endocardial surface which is able to distinguish between normal, ischemic (but viable), or infarcted myocardium (Fig. 14.3). Furthermore it allows accurate manipulation of the injection catheter in the left ventricle and therefore precise transendocardial injection. This allows targeted delivery of cells into specific areas, for example, BMSCs tend to be injected into the peri-infarct zone as it has been shown that BMSCs do not engraft well into scarred myocardium compared to skeletal myoblasts which seem capable of engrafting in fibrotic tissue [73]. The main disadvantages of this system are the additional time and costs required to produce an accurate electromechanical map to guide transendocardial injection together with additional operator training.
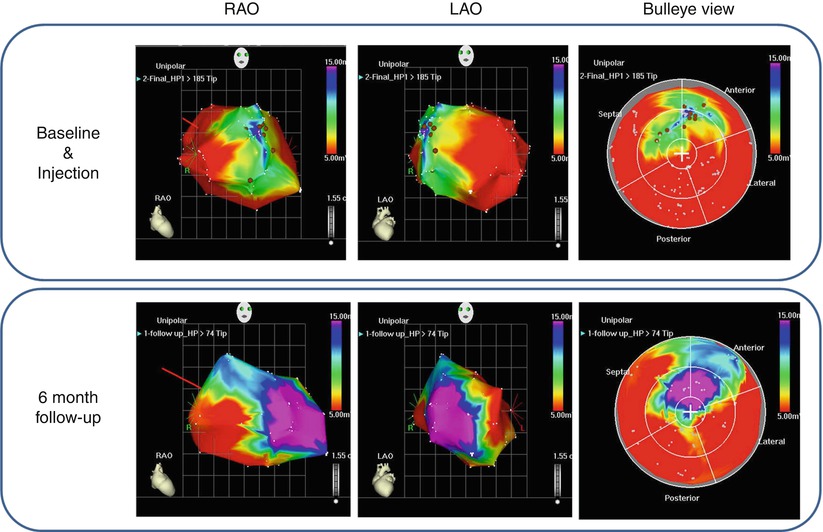
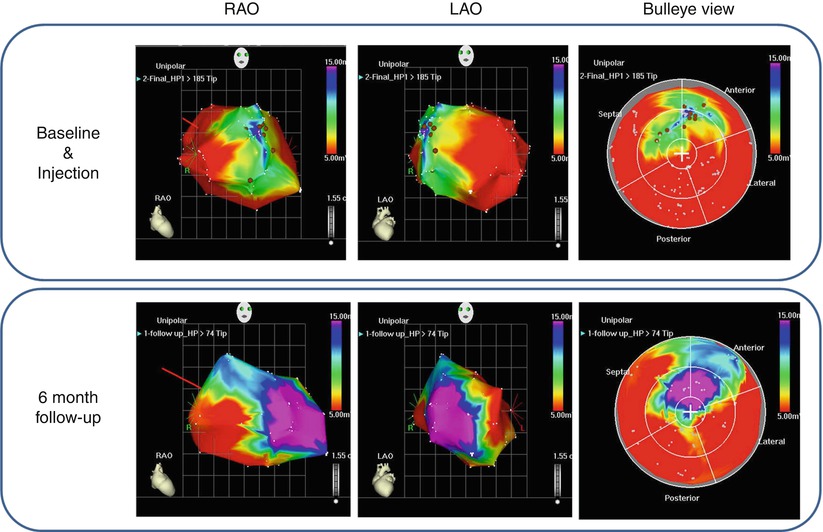
Fig. 14.3
NOGA®-guided injection of bone marrow-derived progenitor/stem cells (BMSCs) using the MyoStar™ catheter in a patient with ischemic cardiomyopathy performed at our institution. The top panel represents baseline NOGA® mapping demonstrating a large area of scar tissue (red, voltage potential <6.9 mV) and hibernating myocardium (green-yellow, voltage potential 7–15 mV) in the anteroseptal regions. The brown spots represent injection sites. The bottom panel represents repeat NOGA® mapping at 6-month follow-up, and there appears to have been an improvement with more viable myocardium present (purple, voltage potential >15 mV)
The MyoStar™ injection catheter (Fig. 14.4) incorporates an integrated injection needle and a location sensor at the tip which interfaces with the NOGA® 3-D mapping system. The 115-cm deflectable tip catheter consists of an outer shaft and inner core lumen, which runs the full length of the catheter and culminates distally in a nitinol 27-gauge needle. The core lumen can be advanced and retracted independently of other catheter movements. The proximal handle contains controls for catheter tip deflection, needle advancement, and the injection port for needle lumen injection. The catheter is prepared by adjusting the retractable needle extension length at 0° and 90° flex, setting the needle length to a maximal needle-to-wall ratio of 0.6. A 1-mL Luer Lock syringe containing the injectate is connected to the injection port, and the catheter “dead space” is filled with the cell suspension prior to introducing the catheter. Multiple injections can be performed at specific sites identified on the 3-D NOGA® electromechanical map – at our institution we perform circumferential 10 × 0.2-ml injections 1–2 cm apart into the hibernating myocardium around scar tissue. Needle injection into the true apex and myocardial segments with wall thickness less than 0.8 mm should be avoided to minimize the risk of perforation. This technology appears to be associated with a low complication rate in the preclinical and clinical studies performed [72, 74].
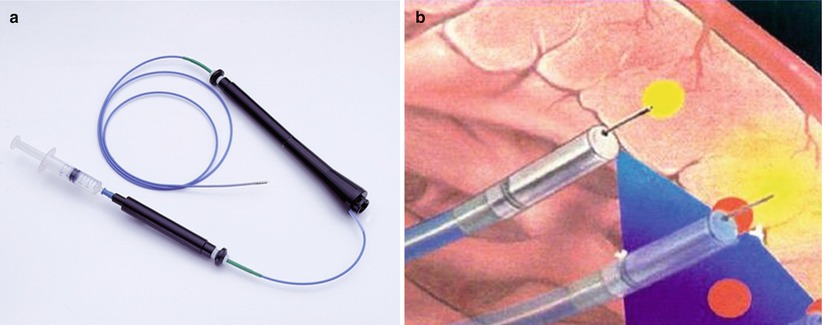
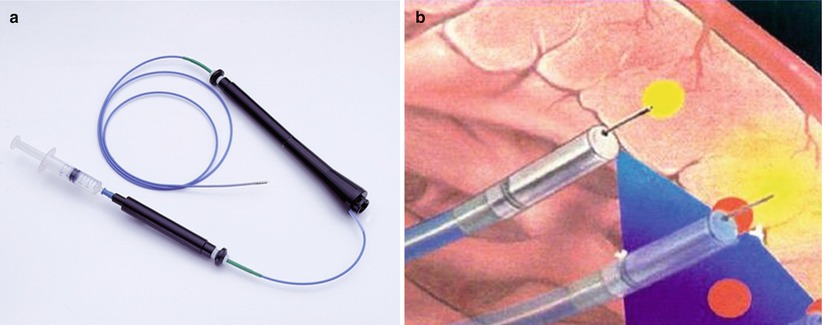
Fig. 14.4
Percutaneous transendocardial injection using the MyoStar™ delivery catheter. (a) A flexible, steerable, injection catheter is advanced retrogradely across the aortic valve into the LV cavity, and injections are guided by electromechanical mapping. (b) The catheter tip incorporates a fine nitinol 27-gauge needle which is normally retracted (Images courtesy of Biosense Webster, Diamond Bar, CA. © Biosense Webster, Inc., 2012)
The MyoCath™ (BioHeart) injection catheter is similar to the MyoStar™ and consists of a 115-cm long deflectable injection catheter that also contains an integrated core lumen with a 25-gauge stainless steel needle at its distal end. This can also be advanced and retracted from the tip of the catheter and provides for multiple injections to a predetermined needle insertion depth. The MyoCath™ is however guided by fluoroscopic guidance, and additional imaging such as transesophageal echocardiography is sometimes utilized.
The StilettoTM (Boston Scientific) catheter (Fig. 14.2d) is also guided fluoroscopically and thus lacks the precision of the NOGA® system although real-time MRI guidance has shown promising results [75]. It contains three separate, independently moveable components: two steerable guiding catheters (9Fr and 7Fr) and an inner spring-loaded needle component. Catheter tip orientation is accomplished by guiding catheter manipulation, and the injection needle is set to a fixed depth (3.5 mm). The spring-loaded advancement mechanism theoretically allows the device to overcome fibrotic scar tissue resistance encountered during needle penetration. This technology is still under investigation; preclinical studies suggest that this is a “safe” technique [76] although higher than expected rates of pericardial effusion has led to the use of the Stiletto™ catheter being halted in the GENASIS investigational trial of VEGF-2 biologic for the treatment of refractory angina.
BioCardia’s Helix™ catheter (Fig. 14.5a) has a small, hollow, distal corkscrew needle, which is rotated into the endocardium to provide active fixation (similar to active fixation pacing leads) and to allow local delivery of biological therapeutic products. This catheter is used in combination with the BioCardia Morph® Deflectable (steerable) Guide Catheter (Fig. 14.5b) which helps catheter navigation within the LV under fluoroscopic guidance only. By eliminating the process of electromechanical mapping, this technique may have advantages in terms of procedural time and cost. Preclinical studies have proven safety and efficacy of this delivery method [77], and there is preliminary data to suggest that this method may be clinically effective [78].
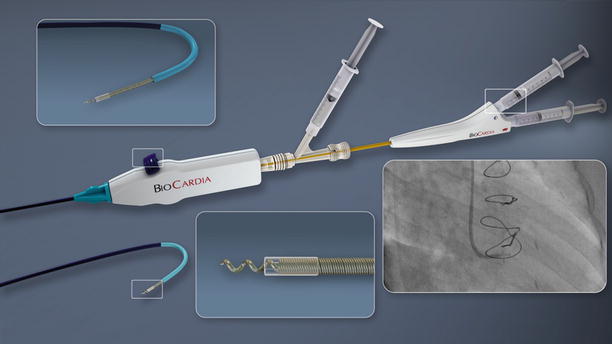
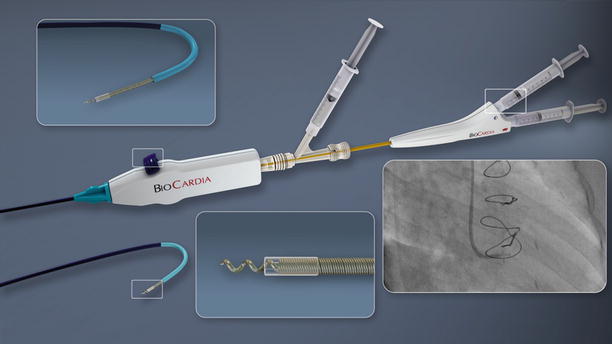
Fig. 14.5
BioCardia Catheter Delivery System. The Helical Infusion Catheter for transendocardial delivery is advanced through the Morph Guide. The therapeutic lumen discharges at the distal tip of the helical needle. A second lumen discharges at the base of the helix for delivering contrast and confirming positioning. The Morph Universal Deflectable Guide Catheter is advanced through the aortic valve over a guidewire and provides the ability to guide the Helical Infusion Catheter (Images courtesy of BioCardia, San Carlos, CA)
Trans-coronary-venous intramyocardial (TVIM) injection can be performed using the Pioneer® catheter system (Medtronic Inc., now the Medtronic Pioneer catheter) that is placed percutaneously into the coronary sinus and target vein (Fig. 14.6). Using incorporated intravascular ultrasound (IVUS) guidance, a 24-gauge nitinol needle is extended to perform transvenous myocardial puncture. A 27-guage microinfusion catheter (IntraLume™) is then advanced though the needle to the targeted areas for cell delivery. Initial studies have confirmed the feasibility and safety of this approach in porcine models [79]. A phase 1 clinical trial has confirmed the safety and efficacy of this delivery method of skeletal myoblasts for the treatment of chronic ischemic LV systolic dysfunction [80]. Furthermore, an animal study demonstrated that the TVIM injection of BM-derived mononuclear cells may lead to a greater retention of the cellular product at the target area as compared to intracoronary infusion [81]. The main limitation to this approach is related to the technical difficulties associated with variation in coronary venous anatomy, which can make it difficult to selectively cannulate the target vein.
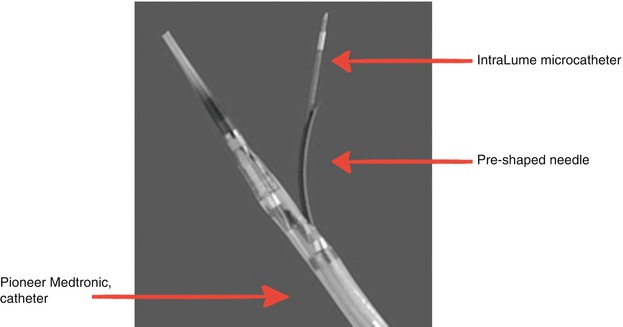
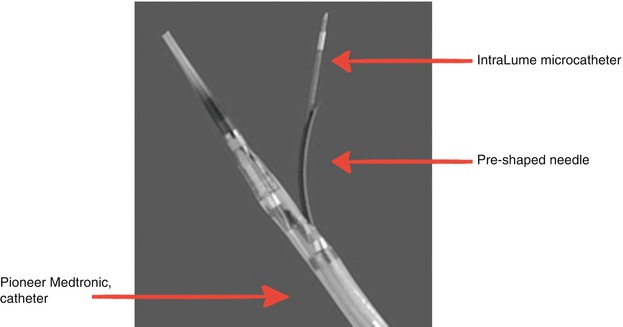
Fig. 14.6
Pioneer (Medtronic, Inc.) catheter tip with IntraLume micro-catheter advanced through the needle tip. IVUS transducer is located at tip of TransAccess catheter
In summary, several techniques have been trialed for cell delivery in patients with acute and chronic ischemic heart disease. In animal models all routes appear to be equally effective in improving cardiac function although the exact mechanisms of action through which cell therapy may achieve this remains unanswered. There has been translation of all the delivery methods from animal models to humans, and to date the intramyocardial route appears to be the most promising in achieving higher levels of cell retention – whether this translates into improved clinical outcomes is yet to be seen.
Percutaneous Cell Therapy in Acute Myocardial Infarction
The existence of endogenous repair mechanisms as well as the concept of adult stem cell plasticity suggests that cardiac repair may be achieved therapeutically in the setting of AMI. The demonstration of the ability of BMSCs to transdifferentiate into cardiomyocytes and improve LV function following AMI in a mouse model [6] has prompted rapid initiation of human clinical trials. The first-in-man procedure [82] was performed just 1 year after publication of the landmark animal study. A 46-year-old man who had been treated with primary stenting of the LAD for anterior wall myocardial infarction underwent bone marrow aspiration, and after 6 days intracoronary reinfusion of BMSCs was performed during low-pressure balloon inflation in the previously stented segment of the LAD. At 10 weeks after the BMSC infusion, the infarct area had been reduced from 24.6 to 15.7 % and ejection fraction had increased by 20–30 %. There has since been an exponential growth in clinical trials in an attempt to confirm these initial exciting findings. In the following section we discuss some of these trials according to the delivery method employed.
Intracoronary Injection of Cells
Intracoronary delivery of cells has been the preferred method of delivery in the clinical setting, and BMSCs have been the predominant cell type investigated (Table 14.2). In the first human trial, Strauer et al. [83] reinfused intracoronary BMSCs 7 days after AMI. This study appeared to confirm the findings of the earlier animal studies with the cell-treated group showing significant improvement in myocardial perfusion and a reduction in the infarct region compared to controls. Subsequently the TOPCARE-AMI investigators randomized patients into intracoronary infusion of BMSCs or ex vivo expanded circulating progenitor cells 4 days after AMI [84]. This resulted in a significant improvement in global and regional LV function in both groups and a beneficial effect on the postinfarction remodelling process manifest by a profound improvement in wall motion abnormalities in the infarct area and a significant reduction in end-systolic LV volume at 4 months post AMI. The LVEF further improved at 12 months resulting in a total increase of 9.3 % at 1 year [98].
Table 14.2
Clinical trials of intracoronary stem cell therapy in acute myocardial infarction
Study name (ref) | Study design | Cell type | n | Timing postinfarction | Follow-up period | Primary outcome |
---|---|---|---|---|---|---|
Strauer et al. [83] (2002) | NRC | BMSC | 20 | 5–9 days | 3 months | Reduction in infarct region from 30 ± 13 to 12 ± 7 %, (P = 0.005), in cell-treated group |
TOPCARE-AMI [84] (2002) | NR | BMSC or PBSC | 20 | 3–6 days | 4 months | Improvement in global LVEF from 51.6 ± 9.6 % to 60.1 ± 8.6 % (P = 0.003) |
Fernandez-Aviles et al. [85] (2004) | NR | BMSC | 20 | 8–18 days | 6 months | Decrease in end-systolic volume, improvement in regional contractility and global LVEF from 51.3 ± 6.6 % to 57.1 ± 10.4 (P = 0.002) |
BOOST [86] (2004) | RCT | BMSC | 60 | 4.8 days | 6 months | Improvement in global LVEF in cell-treated group by 6.7 % compared to 0.7 % in controls (P = 0.0026), but effect was only maintained in large infarcts at long-term follow-up |
Kuethe et al. [87] (2004) | NR | BMSC | 5 | 6 days | 3 months | No improvement in global LVEF |
Bartunek et al. [88] (2005) | NRC | BMSC | 35 | 12 days | 4 months | Reduction in perfusion defect in cell-treated group with improvement in LVEF from 45.0 ± 2.6 % to 52.1 ± 3.5 % (P < 0.05) |
Meluzin et al. [89] (2006) | RCT | BMSC | 66 | 5–9 days | 3 months | Dose-dependent improvement of regional myocardial function and global LVEF |
LEUVEN-AMI [90] (2006) | RCT | BMSC | 69 | 1 day | 4 months | No improvement in global LVEF although there appeared to be significant reduction in myocardial infarct size (BMSC treatment effect 28 %, P = 0.036) |
ASTAMI [91] (2006) | RCT | BMSC | 97 | 6 days | 6 months | No improvement in global LVEF |
REPAIR-AMI [92] (2006) | RCT | BMSC | 204 | 3–7 days | 12 months | Improvement in the global LVEF was significantly greater in the BMSC group than in the placebo group- 5.5 ± 7.3 % vs. 3.0 ± 6.5 % (P = 0.01) |
TCT-STAMI [93] (2006) | RCT | BMSC | 20 | Immediate | 6 months | Improvement in LVEF from 53.8 ± 9.2 % to 58.6 ± 9.9 % (P < 0.05) |
Penicka et al. [94] (2007) | NRC | BMSC | 27 | 9 days | 4 months | No improvement global LVEF |
Tatsumi et al. [95] (2007) | NRC | PBSC | 54 | 3 days | 6 months | Improvement in global LVEF in cell-treated group by 13.4 % compared to 7.4 % in controls (p = 0.037) |
BALANCE [96] (2009) | NRC | BMSC | 124 | 5–9 days | 60 months |