PET and PET/CT in Radiation Oncology
Michael P. Mac Manus
Rodney J. Hicks
Most patients with malignant disease require radiation therapy at some point in their illness. At many institutions, more than one half of all radiation therapy treatments are given with “radical” or curative intent (often described as “definitive” radiation therapy in the United States), although the proportion varies widely by country, treatment center, and by disease type (1). Cure rates for cancers treated primarily with definitive radiation or chemoradiation show wide variation according to disease type and stage. For example, more than 80% of patients with early stage nodular lymphocyte predominant Hodgkin lymphoma may be cured with radiation therapy alone (2), whereas, for anaplastic thyroid cancer, fewer than 5% of patients will survive for 5 years despite aggressive concurrent chemoradiation (3).
Long-term survival rates for most cancers treated with definitive radiation therapy fall between these extremes, and appropriate patient selection is essential to ensure that this potentially life-saving but frequently toxic form of therapy is administered only to those who are most likely to benefit from it. Early relapse with metastatic disease after aggressive local therapy is generally the result of a failure of the staging process to identify the true extent of disease prior to treatment. Patients with a priori incurable disease are generally better served by palliative therapies, which can often include low-dose radiation therapy for local symptom relief.
When curative or definitive radiation therapy is being considered, most often for patients with a solid tumor that is considered unresectable or where radiation offers the best chance of cure with organ preservation, the radiation oncologist needs to know a number of key pieces of information. These include:
Does the patient have distant metastasis that would make curative therapy futile?
Does the patient have nodal disease, and if so, is the disease of an extent that can be treated using radiation with curative intent?
What is the distribution of all gross tumor in three-dimensional space in relation to the anatomy of normal tissues?
This essential information can often be obtained only by accurate imaging, placing radiologists and nuclear medicine physicians squarely at the center of patient selection and treatment planning for many cancers. Of course, other information such as pathology reports, clinical examination, and endoscopy results may provide crucial additional information, depending on the clinical situation, but imaging is usually the foundation for patient selection and treatment planning.
Every physician knows that high doses of ionizing radiation can cause severe toxicity to normal tissues. The risk of significant treatment-related morbidity or even mortality is determined by the intrinsic radiosensitivity of the organs that are irradiated, by the volume of normal tissue exposed to radiation, and by the dose and fractionation of the radiation that is administered. Modern computed tomography (CT)-based radiation therapy treatment planning systems allow accurate estimates of normal tissue irradiation by providing dose-volume histograms for organs at risk and thereby facilitating the production of treatment plans that minimize unnecessary irradiation of normal tissues.
An ideal treatment plan is one that delivers a killing dose of radiation to the tumor but no dose at all to the normal tissues. The closest approximation to this impossible ideal may be achieved theoretically by using a proton beam to deliver therapy (4), but in conventional clinical practice carefully shaped and modulated photon beams can produce highly conformal treatment plans with acceptable levels of normal tissue irradiation.
Accurate treatment planning is dependent on knowledge of the true tumor location in three-dimensional space. A complex and expensive multifield radiotherapy treatment course is a futile exercise if high-dose radiation is not delivered to the entire tumor volume or if the patient is incapacitated by toxicity resulting from unnecessary irradiation of normal tissues. Unfortunately, in the
pre–positron emission tomography (PET) era, many treatments were delivered to volumes that did not include all gross tumor and consequently were doomed to failure from the outset. It is probable that in many of these cases incorporation of PET information into treatment planning might have avoided a catastrophic geographic miss.
pre–positron emission tomography (PET) era, many treatments were delivered to volumes that did not include all gross tumor and consequently were doomed to failure from the outset. It is probable that in many of these cases incorporation of PET information into treatment planning might have avoided a catastrophic geographic miss.
Radiation therapy technology has improved vastly in recent years and will continue to do so in the foreseeable future (5). Our ability to quantify dose to volumes of normal tissues is a significant advance (6) and has been accompanied by an increased ability to treat complex three-dimensional shapes. Linear accelerators with independently controlled multileaf collimators can deliver highly conformal treatments to complex target volumes (7), often making use of complex planning methods such as intensity modulated radiation therapy (IMRT) (8), which enable the radiation oncologist to simultaneously increase tumor dose and reduce normal tissue dose. More sophisticated devices with names like tomotherapy and the Gamma Knife (Elekta Corp, Stockholm) or CyberKnife (Accuray, Sunnyvale, California) deliver highly localized radiation doses, but require very precise image-guided direction. This can lead to improvements in local disease control (9) or facilitate maintenance of a high cure rate with a reduction in toxicity (10), as in some head and neck cancers where parotid-sparing techniques can often avoid permanent xerostomia.
Advances in radiation therapy have increased the need for accurate tumor imaging. The advent of clinical PET, with its vastly superior ability to image many common types of cancer, has coincided with these developments in radiation therapy and has opened up new possibilities for the integration of the best available imaging with the best available radiation therapy. The integration of PET into radiation therapy staging and treatment planning paradigms is becoming increasingly commonplace. Lung cancer is the disease for which PET is best characterized both for patient selection and for treatment planning in radiation therapy patients. Therefore, we will often refer to the literature on lung cancer to illustrate general principles of the use of PET in radiation oncology, but these principles may frequently be applied to other cancers, bearing in mind the significant biological and therapeutic differences that exist between different malignancies. It also must be emphasized that this field is in rapid evolution with extension of PET methods into treatment planning for a broad spectrum of cancers.
PET and PET/CT in Patient Selection for Curative Radiation Therapy
General Principles
For many common cancer types, patient management is determined primarily by the stage of disease at presentation. Demonstration of extensive nodal disease might make surgery inappropriate for a patient and lead to consideration of radical radiation therapy. In turn, demonstration of distant metastatic disease might indicate that radical radiation therapy would be futile. In rare cases, demonstration of a solitary metastasis might lead to an attempt at cure by treating the local and distant disease aggressively.
PET scanning, using fluorine-18-fluorodeoxyglucose ([18F]-FDG) as the tracer, has rapidly acquired a key role in the staging of cancers that are commonly treated with radiation therapy, such as non–small cell lung cancer (NSCLC) (11,12), Hodgkin and non-Hodgkin’s lymphomas (13,14), head and neck cancers, esophageal cancers (15,16), and cervical carcinomas (17,18). FDG-PET is also of clear value in selected patients with high-risk malignant melanoma (19,20), breast cancer, soft tissue sarcoma (21), gastrointestinal cancers (22), and a range of less common neoplasms (23,24).
The more recent advent of PET/CT has been a major advance and is of particular value to radiation oncologists because PET/CT displays metabolic activity denoting active tumor in the context of exquisite anatomical detail. PET/CT images are ideal for clinical staging, as they allow appropriate assignment of focal areas of tracer uptake to the correct anatomical structure, thereby facilitating identification of subtle lymph node and distant metastases.
The PET/CT images themselves are often the best available starting points for radiation therapy planning for FDG-avid tumors, provided the images are captured with appropriate patient positioning. Although markedly superior to PET alone, the advent of PET/CT is an incremental advance on stand-alone PET and does not make the large body of clinical information already obtained with PET or PET plus separately acquired CT any less valuable or relevant.
FDG is currently the most generally useful cancer staging pharmaceutical. However, other PET tracers may be valuable in imaging tumors that have a low uptake of FDG or are located within or near organs with high FDG uptake. For example, [18F]-labeled fluoroethylcholine PET may be effective in imaging prostate cancer in patients planned for high-dose radiation therapy. PET may help to identify lesions that are actively proliferating, using [18F]-labeled fluorothymidine (25) and thereby may distinguish between some inflammatory and neoplastic conditions. This may be of great value when planning radiation therapy for patients with coexistent cancers and inflammatory conditions, such as lung cancer and sarcoidosis, but this warrants further study, as proliferation can occur in nonmalignant tissues as well.
Tumors that are hypoxic tend to be more resistant to radiation and may be identified by PET imaging using [18F]-labeled misonidazole or [18F]-fluoro-azomycin arabinoside (FAZA) as tracers (26). In the future PET hypoxia imaging may be used to help select radioresistant tumors for higher total radiation doses or to guide the delivery of higher doses to hypoxic regions (so-called dose painting) or to identify patients who might benefit from hypoxic cell sensitizers or cytotoxins (26). Despite these interesting exceptions, almost all of the useful clinical trials of PET with relevance to radiation therapy relate to FDG as the tracer.
Much of the strongest evidence that is used to support the use of PET in staging prior to radiation therapy comes from clinicopathological studies of surgical candidates who proceeded to surgical staging after PET. These patients had pathological confirmation of the true tumor extent and nodal involvement and were good tests of the accuracy of preoperative PET. Surgical staging studies have uniformly shown that inclusion of PET in the staging work-up makes preoperative evaluation much more accurate, especially for nodal evaluation of common epithelial malignancies such as lung cancer and esophageal cancer.
There are some dangers in directly extrapolating results from imaging studies of patients with surgical resectable tumors (predominantly early stage with relatively low risk of distant metastasis) to patients who are candidates for radiation therapy for unresectable locoregional disease (more advanced disease with a relatively high risk of distant metastases).
Bayesian principles and indeed the available clinical data both show that the probability of upstaging by PET is greatest in patients already known to have more advanced tumors. Surgical studies may actually underestimate the potential value of PET in patients with more advanced disease. In some neoplasms, such as esophageal carcinoma, small nodes close to an intensely FDG-avid primary tumor may be impossible to visualize on PET due to spill over of activity
from the primary, thereby reducing the sensitivity and specificity of PET for such nodes. For radiation therapy planning this may not be a significant problem because these nodes would routinely be included in the high-dose volume in any case.
from the primary, thereby reducing the sensitivity and specificity of PET for such nodes. For radiation therapy planning this may not be a significant problem because these nodes would routinely be included in the high-dose volume in any case.
Relatively few PET staging studies have been published that have focused on patients with known unresectable disease who were candidates for radical radiation therapy. This is, in part, because pathological confirmation is usually unavailable in patients considered unsuitable for radical surgery, making it difficult to tell if the pretreatment PET was accurate or not. Indeed, invasive staging procedures are usually considered inappropriate and unethical in such patients if they involve any significant risk. Even demonstration of superior accuracy in surgical patients may not be sufficient to prove the utility of PET in radiation oncology patients with more advanced manifestations of the same disease, to the satisfaction of reviewers tasked with advising governments on the utility of new health technology. Some reviewers may inappropriately apply “evidence-based medicine” assessment techniques designed for therapies rather than imaging, demanding surgical staging as “high-level” evidence in nonsurgical patients. Evidence-based medicine is the gold standard only if the most appropriate types of evidence are considered. There may be reluctance to fund PET for radiation therapy patients who have conditions where surgical staging is not usually carried out because of the circular argument that no surgical staging information is available for this particular patient group to prove that PET is more accurate than standard imaging tests. This issue with PET applications in radiation oncology is quite similar to those faced with moving PET forward to clinical practice in tumor staging and treatment response assessment in the past few years, and likely will be increasingly overcome as the performance characteristics of PET are increasingly appreciated.
In a large prospective study of PET staging in patients with NSCLC who were candidates for radical radiation therapy at the Peter MacCallum Cancer Centre, which is discussed further below in the section “Lung Cancer,” the authors attempted to overcome this difficulty by studying survival after PET. The hypothesis of the study was that if PET truly does increase the accuracy of staging, then survival and freedom from progression will be predicted better by a staging work-up that included PET than by conventional staging. In that study the authors found that the overall staging groupings (stage I, II, III, and so forth) determined without PET were a rather poor predictor of survival (27).
Stage groupings determined with the aid of PET were often very different from the CT-based groupings, and PET stage predicted survival much more accurately than CT (P = .0001). These data confirmed that PET could be highly predictive of survival in a large patient population. To show a benefit of PET in such a population, it was irrelevant if the accuracy of any individual lesion was determined by biopsy or not, because the purpose of staging is to predict survival. A confirmatory study was performed at the authors’ center in esophageal cancer, again showing that PET-based stage was a much more accurate predictor of survival than CT-based staging (28).
Detection of Distant Metastasis
Solid tumors that have spread beyond the local lymphatics to involve distant sites are almost always considered incurable with radiation therapy, although in rare cases, such as a solitary brain metastasis, patients may experience long-term survival after aggressive treatment of both primary and metastatic disease. Simply by helping to exclude incurable patients with distant metastasis from futile radiation therapy, PET can apparently achieve better outcomes in those patients who proceed to treatment after PET staging (29). This is an effect of stage migration. PET has the greatest impact in cancers, like lung cancer, where most patients present with disease that already appears locoregionally advanced on conventional imaging. These patients have a high probability of being upstaged to incurable locoregionally advanced or distant metastatic disease by PET.
PET identified distant metastases at a significantly greater rate in patients with mediastinal nodal disease compared to those with apparently earlier stage disease in a series of NSCLC patients from the Peter MacCallum Cancer Centre. The incidence of PET-detected metastasis was 7% in those with apparent stage I disease, 18% in apparent stage II, and 24% in apparent stage III. As the majority of candidates for radical radiation therapy have stage III disease, the impact of PET is greatest in radiation therapy patients compared to surgical candidates who mainly have stage I disease and have a rate of detection of distant metastasis of around 5%. PET-detected metastases were associated with relatively short survival, although those with a single distant metastasis had far superior survival to those with two or more (30).
Lymph Node Staging
Lymph node staging is crucial for many cancers treated with radiation therapy, both for patient selection and for treatment planning. Extensive nodal involvement may be impossible to treat safely or may have such a poor prognosis that aggressive radiation therapy would be futile. Historically, CT has been the most widely used noninvasive method for detecting intrathoracic lymph node metastasis in NSCLC and many other cancers. Survival in NSCLC is powerfully correlated with lymph node staging and drops precipitously when mediastinal nodes contain tumor (25). Because reactive lymphadenopathy is common in lung cancer and because tumor is often present in nodes with a short axis smaller than 1 cm, a commonly used criterion for nodes considered to be positive for tumor, CT has low sensitivity and specificity for the detection of thoracic lymph nodes involved by tumor (31).
The accuracy of FDG-PET in staging the intrathoracic lymph nodes in NSCLC has been directly estimated in numerous clinicopathological studies. In all of these studies (32,33,34,35,36,37), including a meta-analysis (38), PET has been shown to be more accurate than CT for staging the mediastinum. The best noninvasive results have been obtained by correlating the results of both PET and CT images (36). The results of these clinicopathological staging studies are of great importance for radiation oncologists. They conclusively prove that when PET is used in addition to CT to evaluate intrathoracic nodes for malignancy, the accuracy of the assessment is significantly greater than for CT alone.
Although it is impossible to state with certainty that PET is accurate in the assessment of any single node without excisional biopsy, it is clear that in NSCLC, treatment planning based on PET/CT is on average much more likely to be accurate that treatment planning based on CT alone.
Upstaging of nodal disease by PET is also commonly seen in esophageal cancer (39,40), with important implications for radiation therapy when nodes are more distant than the paraesophageal nodal station, as discussed further below, although evaluation for distant metastasis may be even more accurate (41). PET increases the detection rate of gross nodal involvement in locoregionally advanced malignant melanoma (42,43), for which radiation therapy is often an important component of therapy, especially in the head and neck area. However, unlike sentinel node biopsy, PET does not reliably detect microscopic disease (44) in melanoma, and it is therefore rarely useful in stage I or II disease. PET may also provide crucial information on
the nodal status of patients with head and neck cancer (45) or breast cancer (46), with implications for the use of radiation therapy.
the nodal status of patients with head and neck cancer (45) or breast cancer (46), with implications for the use of radiation therapy.
Integration of PET and PET/CT into the Radiation Therapy Planning
Process
Complex three-dimensional radiation therapy is conventionally planned using data acquired from a dedicated CT scanner. This provides both essential three-dimensional anatomic information and a three dimensional electron-density map, crucial for calculating radiation absorption and scatter. Although necessary for dose calculation in radiation oncology, CT by itself often provides insufficient information on the anatomic extent of tumor to allow accurate contouring of disease. To image the boundaries of a neoplastic lesion successfully on CT, there must be sufficient contrast between the lesion and normal tissues.
When tumors have similar imaging characteristics to surrounding normal tissues, as is often the case for lesions in the liver or spleen, they may be completely invisible on CT. Consequently, when CT is used to guide curative radiation therapy, geographic miss and ultimate treatment failure may be inevitable. Failure to image the boundary between tumor and atelectatic lung is a common problem for the radiation oncologist relying on CT for treating lung cancer and may lead to unnecessary irradiation of large volumes of collapsed lung in order to avoid a geographic miss.
As discussed above, accurate lymph node staging is also crucial for treating such locoregionally advanced cancers with curative intent (47,48), and CT scanning is often inadequate because of the unreliability of lymph node size as a predictor of involvement (49). In the absence of any better noninvasive method of staging, for many cancers CT has historically been the nearest thing to a gold standard for radiotherapy planning, albeit a flawed one.
In the years since the introduction of clinical PET, its importance as a tool for radiation therapy planning has become increasingly recognized (50). Manufacturers have become aware of the need to accommodate the importation and display of PET information in radiotherapy planning systems. They have produced systems capable of allowing seamless integration of PET/CT information into the radiation therapy (RT) contouring workstation.
When PET and CT imaging are combined for radiotherapy planning, the aim is to produce a biological target volume (BTV) (51), incorporating all available structural and functional imaging. At the authors’ center, the PET/CT scanner suite has had identical laser positioning devices fitted to those in our simulator and linear accelerator suite, ensuring reproducibility of patient setup for PET/CT, simulation, and treatment. After a treatment planning PET/CT scan is carried out with the patient in the radiotherapy treatment position with appropriate immobilization devices fitted, CT and PET data are imported into the treatment planning software. For centers without PET/CT, separate acquisitions of PET and CT must be carried out with the same rigorous patient positioning.
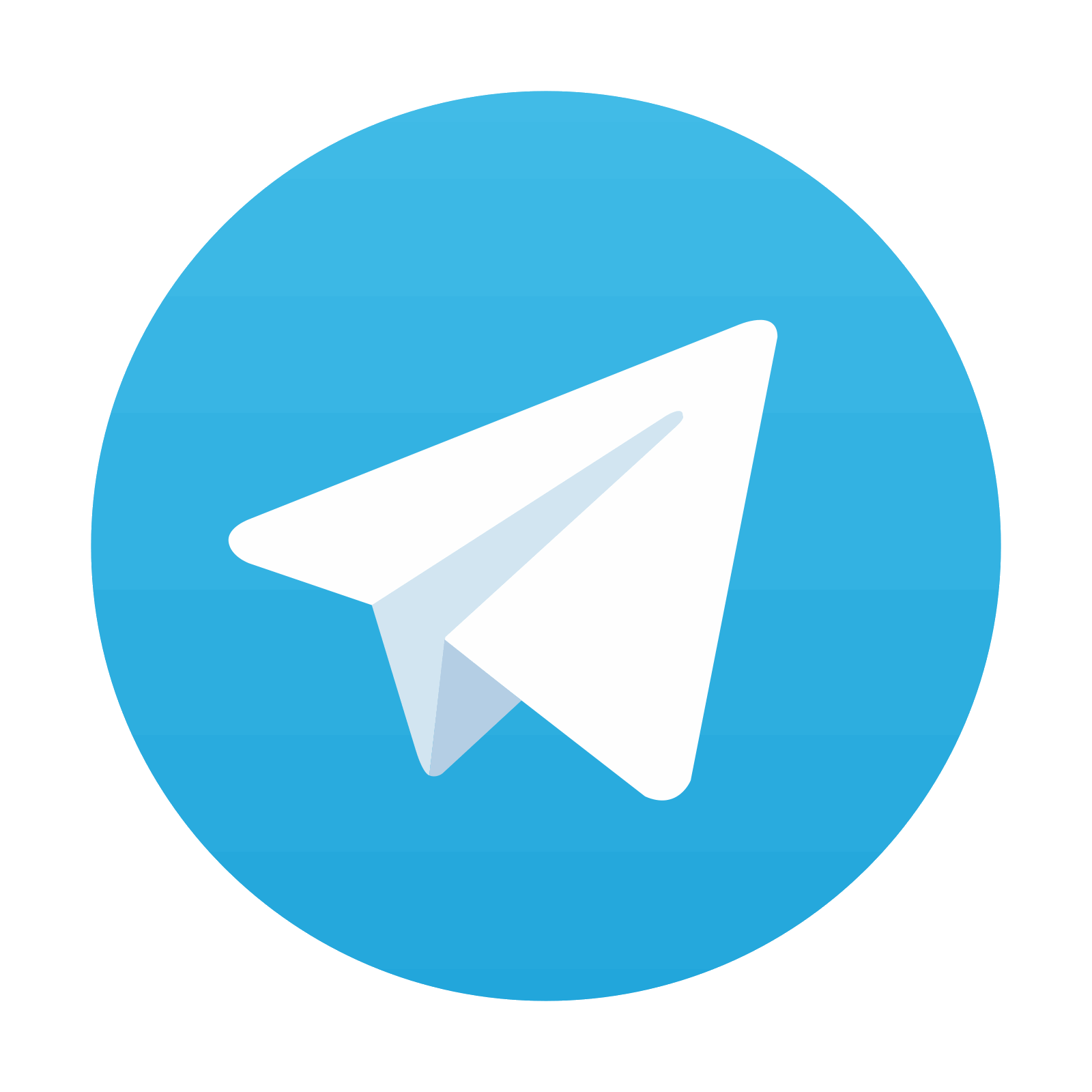
Stay updated, free articles. Join our Telegram channel
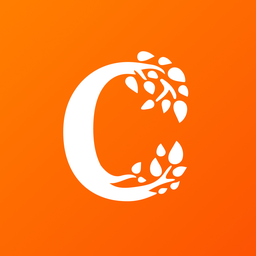
Full access? Get Clinical Tree
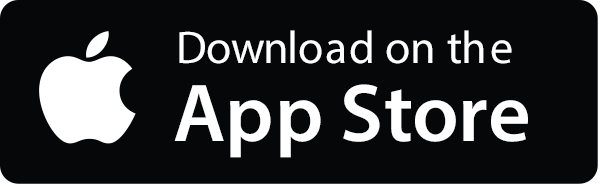
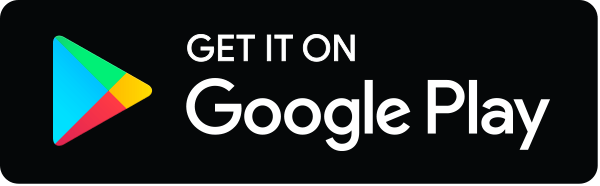