Fig. 9.1
The model implemented in Philips PET/MR system. The two modules are located at adequate far distant apart, minimizing mutual interference between the two systems, and data are acquired in a sequential mode (Image is courtesy of Philip Health Care, Inc.)
The other sequential system was adopted by GE Healthcare (Milwaukee, USA) where two scanners, one hybrid (PET/CT) and one stand-alone (MR), are separated and installed in two different rooms as shown in Fig. 9.2. The two separate systems have the same imaging table that can be transferred from one room to another achieving a multimodality sequential PET/CT and MR diagnostic examination [13]. This system has minimal if no mutual interference between the two scanning units, and therefore image quality and quantitative accuracy are maintained. While this approach provides more flexibility to use the two imaging systems separately and independently and can be optimized to select those patients who need PET/CT or a combination of PET/CT/MR, it remains an additional source of radiation due to CT part of the examination besides likelihood of patient motion that causes undesired imaging artifacts [13].
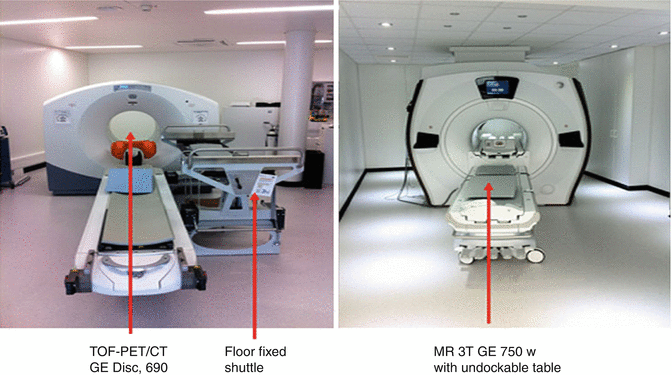
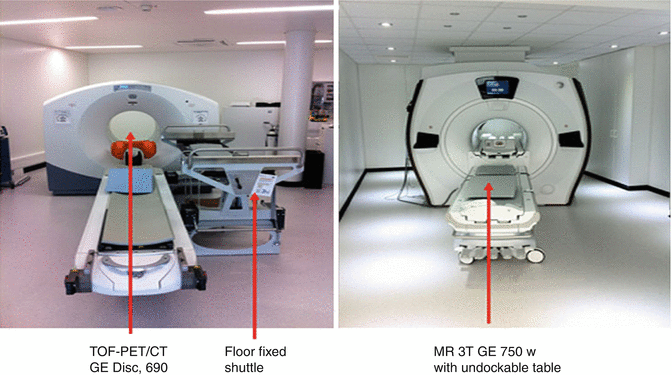
Fig. 9.2
First PET/CT/MR version adopted by GE Healthcare using 3 T MRI system and a TOF-PET/CT in two rooms directly adjacent to each other (Taken from Ref. [14] with permission)
On the other hand, the concurrent PET/MR systems provide a simultaneous data acquisition preserving the temporal and spatial correlation between the two data sets. This approach is either a PET insert located inside the magnet bore or alternatively a PET detector fully integrated into an MR scanner [14–16]. Initial attempts were focused on the insert type to reduce positron range and thus improved spatial resolution of the PET data. However, some challenges are needed to be tackled due to the presence of PET scintillator and associated electronics within the MR field of view. Magnetic susceptibility of the PET components needs to be minimized; otherwise, a deleterious influence on the static field would impact data acquisition and image quality. Therefore, shielding of the magnetic-sensitive components would be essential to maintain field homogeneity and minimize cross talk interference. Alternatively, using magnetic insensitive PET components or placing the PET electronics outside the fringe field would be an alternative option. Size constraints would impact negatively on PET detection sensitivity as the axial extent would be limited as well.
The insert-type PET/MR provides a reduced total acquisition time, minimizes likelihood of patient motion, and doesn’t require major modifications in the MR magnet configurations. The PET insert design is successful in scanning small objects like small animals’ imaging, brain, and extremities but of limited feasibility in whole-body PET/MR due to space limitations that created in the magnet bore. In addition, removing the insert would make the MR to work as a single imaging module providing a cost-effective less integrated option [6, 17].
In comparison to PET/CT, the integrated design is a unique feature that characterizes PET/MR over PET/CT in providing a perfect intrinsic co-registration. Reduction of the total acquisition time is also another advantage provided by simultaneous PET/MR systems in comparison to sequential design. However, integrated design is more challenging and expensive and requires major changes and modification when compared to separate scanning units. The first hybrid whole-body PET/MR scanner (Biograph mMR, Siemens Healthcare) was installed in 2010. It is based on the Siemens Verio 3-T MRI scanner using avalanche photodiode with water cooling mechanism. The bore has been reduced due to this integration from 70 to 60 cm and the PET detector was placed behind the RF coil [14] (Fig. 9.3).
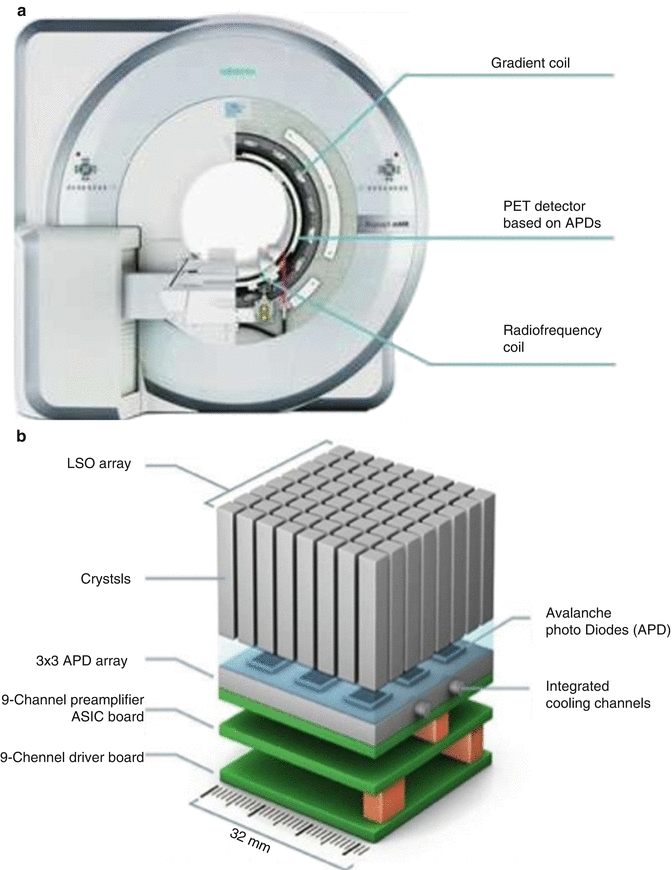
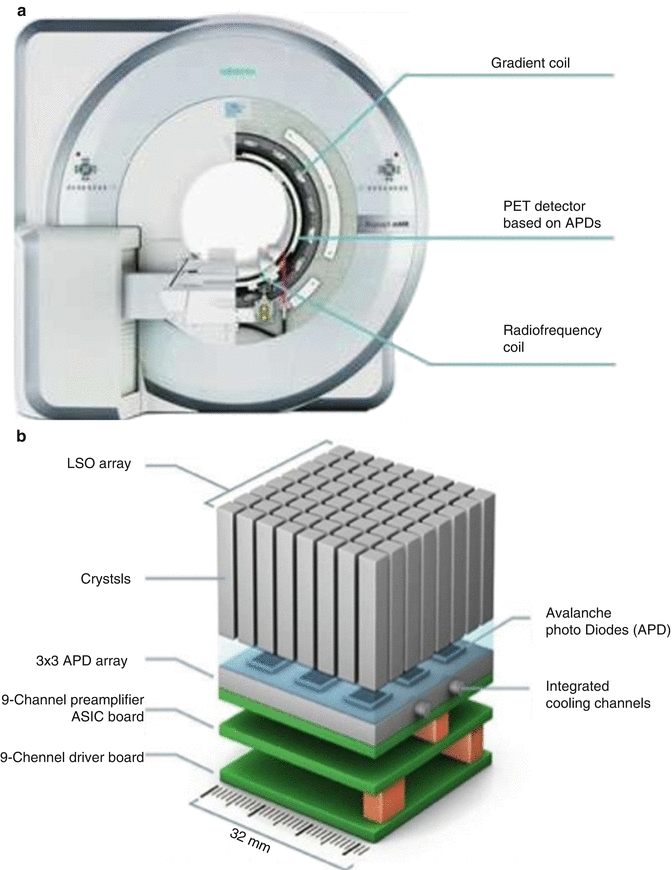
Fig. 9.3
(a) Cross section of the Siemens fully integrated PET/MR (Biograph mMR, Siemens Healthcare, Inc.). (b) PET detector module showing the segmented LSO crystal coupled to APD with the integrated cooling system (Images are courtesy of Siemens Healthcare)
Note that larger-bore magnet is also challenging because the gradient system pays a steep price and performance penalty for its increased size as power requirements go steeply with radius and manufacturing tolerances for gradient shielding become much more demanding [18]. Having the PET detector behind the RF coils allows for reduction of interference with excitation pulses, but temperature and other gamma rays’ attenuation and photon scatter need to be addressed. A split magnet is an approach that also permits PET detector integration and facilitates coupling of the processing electronics, rendering the signal more robust to interference [19].
The other simultaneous PET/MR system recently reported by GE Healthcare is the Signa TOF-PET/MR. The MR component is based on the GE 3-T Discovery 750w MRI system which has an inner bore of 70 cm diameter. The PET scanner has a transverse FOV of 60 cm and an axial extent of 25 cm (89 slices) and comprises of five rings of 112 detector blocks. Each block consists of 4 × 9 array of lutetium based with similar density to LYSO crystal [3.95 mm (transverse) × 5.3 mm (axial) × 25 mm3 (depth)] coupled to 1 × 3 arrays of SiPM devices [20]. The PET detectors’ modules are centered inside the MR gradient set and mounted on the outside of the RF body coil that provides additional space to accommodate the PET detector ring with a 60-cm patient bore. The PET detector relies on SiPM photodetector technology and uses a custom-made ASIC for readout, which permits gain adjustment on the individual pixel level.
Preliminary system performance showed 4-mm spatial resolution (using line source), 10.5 % energy resolution, 22.5 kcps MBq−1 system sensitivity based on NEMA measurements, and 215 kcps at 17 kBq/ml activity concentration [21]. Initial evaluation resulted in roughly more than 50 % of dose reduction can be clinically achieved [22]. The relatively high sensitivity is owing to large axial extent (25 cm), detector depth, and recovery of events occurred by Compton interactions within the detector [23]. The system is shown in Fig. 9.4. The three major vendors of PET/MR systems are summarized in Table 9.1.
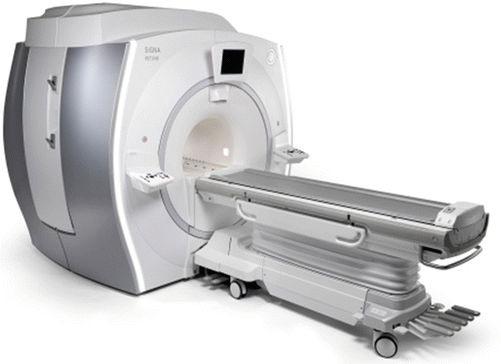
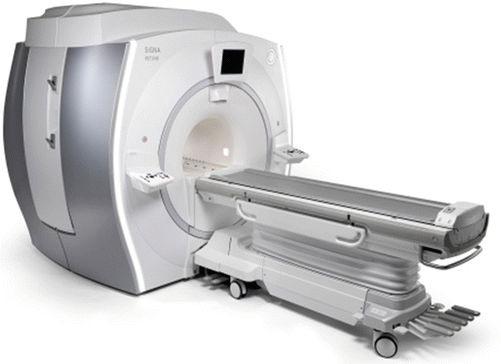
Fig. 9.4
GE Healthcare TOF-PET/MR integrated system (i.e., Signa) that simultaneously acquires PET and MR signals. It combines simultaneous data acquisition from GE’s latest 3.0 T MR750w and SiPM-based PET technology
Table 9.1
Summary of the PET/MR systems released by the three major manufacturers
PET/MR system | Biograph mMR (Siemens) | Ingenuity TF (Philips) | Discovery PET/CT 690 + MR 750 (GE) |
---|---|---|---|
PET system | |||
Crystal material | LSO | LYSO | LYSO |
Crystal elements dimension | 4 × 4 × 20 mm3 | 4 × 4 × 22 mm3 | 4.7 × 6.3 × 25 mm3 |
Photomultipliers | No, 4032 APDs | 420 | 1024 |
Ring diameter | 65.6 cm | 90.3 cm | 88.6 cm |
Transaxial FoV | 59.4 cm | 67.6 cm | 70 cm |
Axial FoV | 25.8 cm | 18.0 cm | 15.7 cm |
Energy window | 430–610 keV | 460–665 keV | 425–650 keV |
Coincidence window | 5.9 ns | 6 ns | 4.9 ns |
Time of flight | No | Yes | Yes |
MR system | |||
Field strength | 3 Tesla | 3 Tesla | 3 Tesla |
Bore diameter | 60 cm | 60 cm | 60 cm |
Max FoV | 50 × 50 × 45 cm3 | 50 × 50 × 45 cm3 | 48 × 48 × 48 cm3 |
Field homogeneity (40 cm3) | 0.25 ppm | 0.5 ppm | 0.25 ppm |
PET/MR | |||
Acquisition | Simultaneous | Sequential (same room) | Sequential (2 separate rooms) |
PET attenuation correction | MR-based | MR-based | CT-based |
9.3.1 PET/MR Technical Challenges
Allocation of the PET detector within the magnetic bore and removing mutual interference with MRI components are the two initial but major issues that need to be tackled before realizing an integrated hybrid PET/MR system. The magnetic field has its well-known degrading effect on the photomultiplier tube (PMT). The vacuum of the PMT has several accelerating dynodes of varying potential difference. The photoelectrons emitted from the tube cathode are amplified through this process which is very sensitive to magnetic fields. The existence of PMT-based PET detector in the MRI magnetic fields results in a potential signal deterioration and distorted PET image. Another undesired feature of the PMT is the bulky structure that doesn’t facilitate an easy integration inside the magnet core.
The strong static magnetic field and abrupt changes in the gradient magnetic field in addition to the radio-frequency pulse are major MRI elements that influence the PET detector system. Furthermore, the radio frequency and its rapidly changing pulses create eddy currents in any conductive material present in the field in addition to mechanical and vibrational noise [25–27]. Eddy current is a phenomenon created when a time-varying magnetic flux through a closed circuit produces an electromotive force proportional to its rate of change in a close proximity conductive material. It results from the excitation RF pulse and the rapidly changing gradient coil and could have several imaging drawbacks like a spatially variant phase change of the resonance signal, or the associated field will alter the spatial encoding of the signal leading to a distortion of the reconstructed images [24].
The presence of PET electronics in the magnetic field of the MR system has also its own drawbacks. It is important to maintain the magnetic field homogeneity avoiding any source that affects this uniform pattern. PET electronics including crystal, front-end electronics, any RF or radiation shielding, and particularly ferromagnetic components could potentially affect field homogeneity and linearity of the coil gradient system. Therefore, the shielding material should be carefully selected and optimized for data acquisition and elimination of imaging artifacts and data distortion [28].
In terms of image quantitation, attenuation correction is one of the major challenges that need to be properly tackled in order to achieve reliable (absolute or relative) quantitative measures. Other aspects that are tightly associated with MR imaging are the presence of metallic components and bony structures that have no significant information on the MR images. MR hardware is also critical in photon attenuation and could compromise correction techniques. These points will be discussed later.
9.3.2 What Is the Solution Then?
To solve the aforementioned problems, there were two major but different approaches that were taken into consideration. One of them was to keep the PMT at adequate distance away from the MR interfering signal. The distance was calculated such that the fringe magnetic field drops below 10 mT and the scintillation light was transmitted through lines of optical fibers. The first simultaneous preclinical prototype was built analogous to that way and consisted of a ring of forty eight 2 × 2 × 10 mm3 lutetium oxyorthosilicate (LSO) crystals of 38-mm inner diameter, implementing a one-to-one pixel coupling to three multichannel PMTs (MC-PMT) connected via (2 mm diameter and 4 m long) double clad optical fibers [29].
Shielding the PMT using steel or mu-metal is effective in weak magnetic field but fails in the strong fields used in MRI imaging systems. Using long optical fibers was not a perfect solution and showed some drawbacks such as loss of light signal (five to tenfold), deterioration of energy resolution, as well as degradation of timing resolution [30]. However, recent work on this area has also been extended to use a 90° light guide to transfer the light from the crystal to the optical fibers permitting the PMT to be placed in a magnetic-shielded region of 0.3 mT behind the yoke [31]. A reduction of light loss was achieved by newly developed flexible optical fiber bundle-based block detectors, employing them in a high-resolution integrated PET∕MRI system [31].
The other solution was to use solid-state semiconductor photodetector such as avalanche photodiode (APD) or silicon photomultiplier (SiPM) that are insensitive to high magnetic field of the MR scanner [32, 33]. Avalanche photodiodes can operate in MR magnetic field without apparent distortion and have been successfully coupled to bismuth germinate (BGO) or cerium-doped lutetium oxyorthosilicate (LSO) crystals. The size of the APD is very small, and thus a compact PET/MR design can be manufactured using this type of solid-state photodetectors. The timing resolution in the range of nanoseconds and thus TOF is not possible. However, SiPM has been emerging as a potential and better candidate due to its signal-to-noise (SNR) ratio, higher gain, and timing resolution in the range of sub-nanosecond, and thus time of flight can be realized [34]. Further details on those types of photodetectors are discussed in Sect. 9.2.5.
9.3.3 PET Detector in PET/MR
The current generation of PET imaging systems relies on the full ring design that provides a simultaneous data acquisition of all projection angles at the same time. So detector rotation is eliminated and count sensitivity is greatly improved in comparison to older versions such as partial ring or single ring design. As discussed in Chap. 8, the block detector is the conventional and most adopted standard detector design used in PET imaging scanners. The scintillator block is viewed from the backside normally by 4 PMT that read out the light signal converting it into electric current for further processing and event spatial, temporal, and energy determination.
TOF-PET scanners do utilize the new advances in scintillation crystals, electronics, and computer technology by exploiting the full potential of PET in improving SNR [35]. TOF was not the only feature, but in the last decade, there were several developments in PET imaging such as an improved detector technology, electronic circuitry, data acquisition boards, processing and computer workstations, image reconstruction, and modeling of image-degrading factors, and all of these resulted in overall improved system performance and diagnostic accuracy.
9.3.4 Crystal
Scintillation crystal is the first component that receives the coincidence photons during the scanning process. The crystal role is to shift the wavelength of the incident energy into higher values that permit further downstream signal processing. This is because the light quanta emitted from the crystal have lower energy (higher wavelength) than the incident radiation beam. BGO crystal was the conventional detector material used for many years in PET systems due to its efficient stopping power and nonhygroscopic properties in comparison to Na(Tl) crystal. The scintillation crystal in PET/MR system should be carefully selected so that any magnetic susceptibility must be avoided (i.e., the degree of magnetization of a material in response to an applied magnetic field) [36]. BGO, LSO, and LYSO crystals were found to have lower magnetic susceptibility, but GSO and LGSO are not appropriate and can cause MRI artifacts. The former group is the most common in the current PET/CT and PET/MR systems. Currently, the most preferred crystal type is the LSO-type crystals due to high light output, good detection efficiency, and short decay constant that permits TOF applications.
9.3.5 Photodetectors
9.3.5.1 Photomultiplier Tube (PMT)
The most commonly used photosensors in gamma camera and PET imaging systems are the PMTs due to its high gain that lie in the range of 105–107 in addition to the fast timing characteristics. It is manufactured in wide range of geometries, sizes, and designed base on vacuum tube technology that improved significantly over the years. Some of these improvements are better timing performance, enhanced quantum efficiency, and developments of multichannel and position-sensitive PMT [34]. PMT has two major undesired properties that make its integration in PET/MR systems indeed difficult. These are the sensitivity to magnetic field and the relatively large structure that impedes its easy integration inside the magnetic bore. Keeping the PMT tube at a distance from the magnetic field fringe below 500 μT has been reported in simultaneous preclinical PET/MR system. However, this configuration results in great reduction of scintillation light (90 %) leading to a degraded energy resolution [31].
9.3.5.2 Avalanche Photodiode (APD)
One of the major transitions in the field of PET/MR detector technology is the use of semiconductor photosensors in PET detector design [32]. APD operates below the breakdown voltage, and the signal produced is proportional to the ion pair created and to the energy deposited by the coincident photons interacted with the crystal. APDs also have an advantage over PMTs in not requiring high bias voltages to operate. At reverse bias, a volume close to the junction is depleted of free charge carriers. The charge carriers created in the depleted region drift in the electric field toward corresponding electrodes and, while traversing this region, acquire enough energy to produce electron-hole pairs by impact ionization. The newly created charge carriers may create new ones and so on. Thus, there is an avalanche of electrons and holes moving through the detector. An external circuit then detects these current pulses [37].
The quantum efficiency reaches 70–80 %, and when coupled to LSO crystal (peak wavelength 420 nm), the timing resolution lies in the range of few nanosecond. Avalanche photodiodes provide great advantages in terms of dimension and subsequent detector design compactness. The small size provided by APD is a positive characteristic toward an improved spatial resolution that matches small crystal dimensions and high packing fraction. The insensitivity to magnetic field was central property that made APD an acceptable alternative to PMT in PET/MR systems [38]. APD coupled to LSO crystal in preclinical 9.4 T magnet showed stable performance with no dependence in gain and energy resolution (14.4 % at 511 keV) of APD on the magnetic field. There was also no effect of changes in the orientation of the APD electric field with respect to the main magnetic field lines (parallel vs. perpendicular), hence proving the feasibility of operating such a PET detector module inside an MRI [39].
However, APD has a number of limitations that requires special attention in the detector system. It has low internal gain (102–103) and requires charge-sensitive preamplifiers to convert electron charge to a measurable voltage signal. Charge integration occurs over several nanoseconds to achieve high conversion factors, and pulse pileup takes place at high counting rates. APD needs advanced front-end electronics to meet the lower gain, and therefore special and application-specific integrated circuits are used [40]. Noise and time variation in electron drift during multiplication process are factors that restrict using the APD in time of flight applications [41]. The gain is sensitive to changes in temperature and voltages applied, and thus special measures are required to control these technical factors. Thus, temperature sensitivity of APD imposes special constraint on the design of PET/MR such that a well control over the solid-state detector should be maintained and eddy current, if not minimized, would affect the gain of the electron avalanche due to increased collision with semiconductor lattice [36, 37]; see Table 9.2.
APD can be coupled directly to the scintillator but also can be located at a short distance using optical fibers, depending in large part on system configuration including space allocated for PET module inside the magnet and minimizing the interference between the two subsystems [15, 42]. The advent of APD to the medical arena has been attractive for large academic institutions to build their own hybrid PET/MR systems. It has been successful in initial attempts made to construct simultaneous hybrid systems with further investigations performed to optimize the design and improve the quality of either systems [43]. The first insert-type PET detector was a prototype released by Siemens (BrainPET, Siemens Healthcare, Inc.) providing an opportunity to acquire a simultaneous PET/MR on human brain [17, 43] as mentioned earlier. The insert was integrated with a standard 3-T MRI scanner (Magnetom TIM Trio; Siemens Healthcare, Inc.), and proof-of-principle simultaneous data acquisition was demonstrated. In addition, the BrainPET can be docked at the back of the magnet without obstructing the bore so that the MR scanner can be used in stand-alone mode [17, 43].
Table 9.2
Comparison of three major photodetectors used in clinical or preclinical PET/MR imaging systemsa
Characteristic | PMT | APD | SiPM |
---|---|---|---|
Active area (mm2) | 1–2000 cm2 | 1–100 mm2 | 1–10 mm3 |
Gain | 105–107 | 100 | 105–107 |
Rise time | <1 ns | 2–3 ns | ~1 ns |
Dark current/count rate | <0.1 nA/cm2 | 1–10 nA/mm2 | 0.1–1 MHz/mm2 |
Capacitance (pF/mm2) | 9 | 2–10 | >30 |
Quantum efficiency @ 420 nm (%) | 25 | 60–80 | <40a [~25–75] Photon detection efficiency |
After pulsing | Yes | No | Yes |
Bias voltage (V) | 1000–2000 | ~200–1500 | ~50 [30–80] |
Power consumption | 100 mW/ch | 10 μW/mm2 | <50 μW/mm2 |
Temperature coefficient (%/°C) | <1 %/°C | 2–3 %/°C | 3–5 %/°C |
Bias coefficient | <1 %/V | 10 %/V | ~100 %/V |
Magnetic susceptibility | Very high (mT) | No (up to 9.4 T) | No (up to 15 T) |
Price/channel ($) in 2010 | >200 | ~100 | ~50 |
9.3.5.3 Silicon Photomultiplier (SiPM)
Geiger-mode avalanche photodiode (AVP) or SiPM (also called multi-pixel photon counter) is made of arrays of small APD (e.g., 50 × 50 μm) and operated beyond the voltage breakdown in the Geiger counting mode [44]. The compact size permits also an easy incorporation in small animal PET/MR systems and implementation in depth of interaction-based scanners [31]. In this solid-state photodetector, the high electric field in a very small space that collects the ion pair allows for better quantum efficiency and provides good resistance to magnetic field in contrary to those effects seen in PMT. It was shown that SiPM can tolerate a magnetic field of 7 T [45]. Coupling of the SiPM to scintillation needs some attention especially one-to-one coupling as it increases the number of photodetector in the magnet substantially; however, it eliminates light sharing and improves spatial resolution [31, 41].
Like APD, low temperature is also an important prerequisite for operating SiPM, and variation in temperature can lead to alteration of the breakdown voltage and subsequent variation of photodetection efficiency, dark current, and gain [27]. Each cell in SiPM photodetector works independently, and the charge created from the ion pair is no longer proportional to the energy deposited, but the summed output from all cells is proportional to the energy deposited in the scintillator. It is therefore important to maintain good energy linearity as photodetector outputs need to be compared in crystal identification schemes [11]. While the quantum efficiency of APD is nearly 80 % (as mentioned above), the photodetection efficiency of SiPM is below 40 % when coupled to LSO crystal. Proper eddy current management, cooling system, and accounting for residual inaccuracies in system calibration are measures taken to tackle heat generation and associated problems.
Based on the above discussion, the SiPM has a high gain similar to that revealed from PMT, resistance to high magnetic field, and low bias voltage when compared to APD. The emerging digital SiPMs have shown improved performance and low temperature sensitivity. It also provides a good timing, energy and spatial resolution, as well as good temperature stability, making it a promising candidate concerning their MR compatibility. However, they tend to generate digital electromagnetic noise patterns which might degrade the MR image quality; a design measure that needs to be taken into consideration [46]. Table 9.1 compares the three commonly used photodetectors used in PET/MR.
As previously mentioned, the Signa PET/MR from GE Healthcare utilizes the SiPM technology. This new digital detector is characterized by its enhanced sensitivity; it is up to three times more sensitive than GE Healthcare’s Discovery 690 PET/CT. SiPM photosensor is also being adopted in the European Hyperimage collaboration to build a small animal PET insert inside a clinical 3 T MR system of a ring diameter of 20 cm and axial field of view of 9 cm. Each detector comprises a 22 by 22 array of 1.3 × 1.3 × 10 mm3 LYSO crystals coupled to an 8 × 8 array of 4 × 4 mm2 SiPM where the signal is immediately digitized using application-specific integrated circuit (ASIC) that release digital energy, timing, and channel information [46, 47]. This last feature is substantial as it reduces and could eliminate the interference that affects connection and transmission cables between photodetectors and signal processing circuitry. See Fig. 9.5.
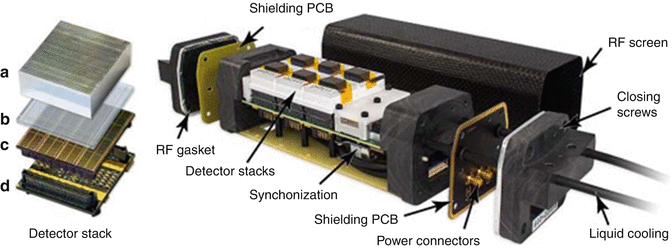
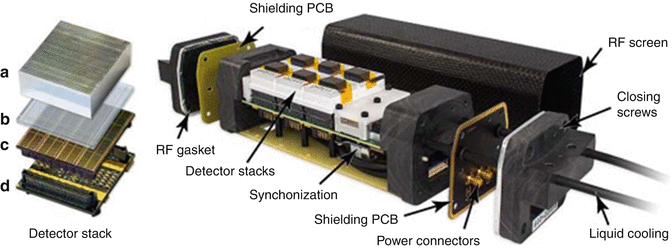
Fig. 9.5
Digital silicon photomultiplier (dSiPM)-based detector stack (left) with scintillation crystal array (a), light guide (b), sensor (c), and interface board (d). On the right side of the figure, the single detection module (SDM) contains up to six detector stacks. The carbon fiber shield is pushed over the module and the RF screen is closed on both sides by shielding plates (From Ref. [48] under Creative Commons Attribution 3.0 License)
9.4 MR System Technology
An MR imaging system has several major components that interplay to acquire an image. The most important parts are static magnetic field, gradient magnet, and radio-frequency coil. The magnetic field magnetizes the human body once the patient is inside the magnet bore. Most of the imaging interest in MR is the hydrogen atom (i.e., protons) due to its plenty and large abundance in human tissues and cells. Under the strong magnetic field applied by the static magnet, the protons are aligned into two directions: parallel and antiparallel. Majority of protons are aligned in parallel with the magnetic field (Bo) in comparison to those protons aligned antiparallel as the former is a lower energy state compared to high energy state of the antiparallel direction. A net magnetization is therefore produced in parallel to the magnetic field (Bo) and takes place along the z-direction. In addition, a precession or rotational motion of the protons is created that precess with frequency proportional to the magnetic field. In clinical setting, 1.5 and 3 T static magnet is used, but higher strength (e.g., 7 and 9.4 T) is used for more advanced application. It is important to maintain the homogeneity of the static magnetic field, and any component of magnetic susceptibility (a property of a material to be magnetic when exposed to magnetic field) must be avoided or adequately isolated within the field of view to keep image quality with minimal distortion.
The gradient coil has an important role in providing a spatial localization of the MR signal by varying the static field strength in the three orthogonal planes in a linear fashion. Gradients are loops of wire or thin conductive sheets on a cylindrical shell lying just inside the bore of an MR scanner. When current is passed through these coils, a secondary magnetic field is created. This gradient field slightly distorts the main magnetic field in a predictable pattern, causing the resonance frequency of protons to vary as a function of position. The primary function of gradients, therefore, is to allow a spatial encoding of the MR signal. Gradients are also critical for a wide range of “physiologic” techniques, such as MR angiography, diffusion, and perfusion imaging [49].
The second important part is the transmission coil which sends uniform radio-frequency excitation at the Larmor frequency over the field of view. Local receive coil or surface coil is placed over the region of interest to improve the magnetic sensitivity and hence signal-to-noise ratio. This coil is also affected by distance such that the sensitivity decreases appreciably beyond a distance equal to the diameter of the coil. Upon relaxation and switching off the RF signal, the information received is processed using a reconstruction algorithm from 2D to 3D images of the resonance signal [27].
Most commercial MRI scanners are superconducting high-field strength magnets because of their faster scanning ability, higher magnetic field homogeneity, higher SNR, and wider range of applications. In a superconducting magnet, a magnetic field is generated by a current that runs through a loop of wire. The wire is surrounded with a coolant, such as liquid helium, to markedly reduce the electric resistance of the wire. Once a system is energized, it maintains its magnetic field [50]. Early work on PET/MR systems was specially focused on manufacturing an MR-compatible PET detector with modifications made to the PET detector system. Recent approaches taken are to modify aspects of the MRI in order to achieve close integration and minimize interactions between the two systems within the magnet bore [25].
9.4.1 Magnetic Shielding
The presence of PET front-end electronics and data transmission cables inside the magnet bore represents one of the major challenges in hybrid simultaneous PET/MR systems. A proper but effective shielding system should be implemented to reduce to an acceptable level the interferences that come from the gradient as well as radio-frequency pulse and able not to disturb the main magnetic field uniformity [51]. Signal reflection and absorption together with material skin depth are important physical measures of the shield. Moreover, magnetic susceptibility, gamma rays’ attenuation coefficient, and eddy currents are factors to be considered in the candidate shield material. Connection and transmission cables need also a sort of attention as it needs to be masked from the above interferences sources.
Reflection loss is proportional to material conductivity and inversely proportional to permeability, while absorption loss is proportional to conductivity, permeability, and thickness of the shield [27]. In electromagnetism, permeability is the measure of the ability of a material to support the formation of a magnetic field within itself. Hence, it is the degree of magnetization that a material obtains in response to an applied magnetic field. Skin depth, however, is inversely proportional to permeability, electrical conductivity, and signal frequency. One skin depth is defined as the required thickness of a metal to reduce the RF to 37 % of its original strength. The smaller the skin depth, the thinner the material is used for shielding to a particular level. Gradient switching typical in MRI sequences can result in count losses in the particular PET detector design studied. Moreover, the magnitude of this effect depends on the location of the detector within the magnet bore and which MRI gradient is being switched. This information is substantial in the design of PET shielding in MR environment [43].
Aluminum (Al), copper (Cu), and carbon (C) are different materials that can be used for shielding. High conductive materials, e.g., copper, show excellent radio-frequency (RF) shielding properties, but have negative impact on the MRI image quality due to induced eddy currents. However, carbon fiber composites are less conductive for low frequencies and thus can minimize MRI gradient-induced eddy currents. Furthermore, they show good RF shielding properties for higher frequencies. First prototypes using carbon fiber have shown excellent eddy current performance, good RF shielding properties, and superior mechanical robustness in a preclinical simultaneous PET/MRI insert in a clinical 3-T scanner [52].
In a different setting of 7-T PET/MR preclinical scanner (RF at Larmor frequency of 300 MHz, 81 kHz from gradient power supply), placing the PET detector module between two carbon fiber tubes and grounding the inner carbon fiber tube to the PET detector module ground reduced the RF and the eddy currents. Further reductions were achieved by adding thin copper (Cu) foil on the outer carbon fiber case and electrically grounding the PET detector module so that all three components had a common ground [43].
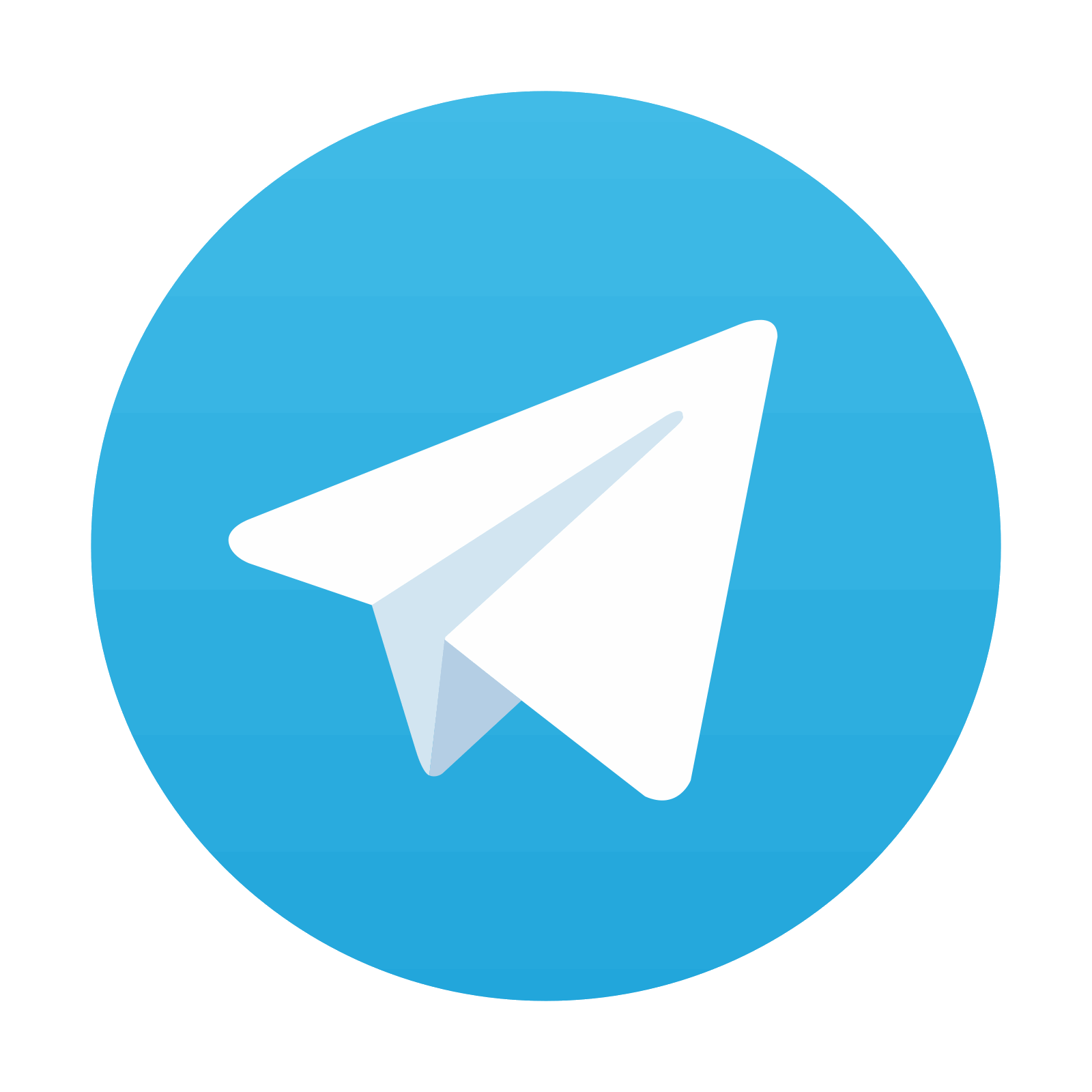
Stay updated, free articles. Join our Telegram channel
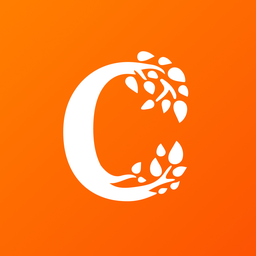
Full access? Get Clinical Tree
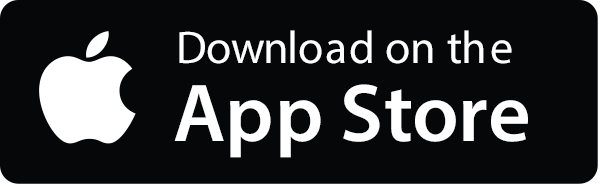
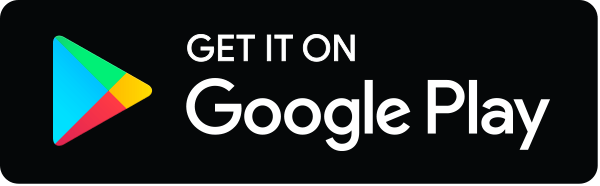