, Valery Kornienko2 and Igor Pronin2
(1)
N.N. Blockhin Russian Cancer Research Center, Moscow, Russia
(2)
N.N. Burdenko National Scientific and Practical Center for Neurosurgery, Moscow, Russia
A distinctive feature of radionuclide methods, including positron emission tomography (PET), is their initial focus on a visual and quantitative assessment of biological processes within the cell (Shinoura et al. 1997; Chen 2007; Kumar et al. 2010; Granov and Tiutin 2013). Thus, if MR or CT perfusion indirectly suggests a degree of malignancy or viability of the tumor tissue by its structural characteristics or hemodynamic parameters, PET with 18F-choline, for example, estimates the intensity of the formation of cell membranes, including endothelial cells, long before the formation of the abnormal vasculature. An assessment of biochemical processes in the brain is of particular interest both in the differential diagnosis of intracranial tumors and in the differentiation of therapy-induced residual tumor necrosis and edema (Dolgushin 2008). Important is the fact that, in PET studies, a metal or other foreign bodies do not give artifacts in images, in contrast to CT or MRI, and their presence in patients is not a contraindication for the study.
Positron emission tomography (PET) using a variety of radiopharmaceuticals (RP) provides unique information about the functional state of tumors by a number of biological processes, such as glucose metabolism, protein/DNA synthesis, rate of cell membrane synthesis, angiogenesis, hypoxia, etc. (Weidner 1995; Hara et al. 1997). The first PET studies with 18F-FDG were conducted on the brain and were directed to the study of functional disorders in dementia, epilepsy, Parkinson’s disease, and Alzheimer’s disease. However, the use of PET with 18F-FDG in brain tumors is limited because of the intense physiological accumulation of the drug in the gray matter. The isotopes that emit positrons generally have expressed specific radioactivity, and, as a rule, only a small amount of RP is needed to obtain the necessary information. The relatively short half-life of the isotopes used in PET helps reduce the radiation dose and allows for repeated measurements at short intervals. Due to the specifics of the primary diagnosis of brain tumors, PET with 18F-FDG is inefficient as compared to MRI. However, 18F-FDG helps visualize tumors with high metabolic activity, such as metastases, glioblastoma, some types of meningiomas, and gray matter lesions, resulting in a decrease in metabolic activity. A simultaneous scanning of the “whole body” and brain with 18F-FDG PET significantly reduces the information value of the study because of the high background RP accumulation in the brain deteriorating the relative contrast of extracranial lesions. This is why the so-called “whole-body” study with 18F-FDG is limited to the level of the skull base.
PET diagnosis identifies tissues by varying degrees of glucose consumption. Since most tumors have a higher metabolism than intact tissues and the glucose consumption by the tumor tissue is higher, PET images display areas of abnormally high tissue metabolism. On the scans, there are foci with increased accumulation of glucose labeled with the isotope 18F. The detection of increased accumulation areas on the background of areas with the normal RP accumulation indicates an increase in metabolic processes in this area, which may be caused by a tumor, inflammation, etc. An area with enhanced metabolism allows to judge with high reliability about the presence and extent of a tumor. In addition to determining the extent of the tumor process and the early detection of its manifestations due to progression, evaluating the effectiveness of cancer treatment, diagnosis of iatrogenic (postoperative) damage, and post-radiation scarring, PET is effective in the differential diagnosis of malignant tumors from benign tumors and non-tumor diseases.
10.1 Radiopharmaceuticals (RP) for PET and the PET Facility Structure
Obtaining RP is a knowledge-intensive, technologically complex, and expensive procedure, which is carried out in specialized laboratory units. The main machines for the production of radionuclides for nuclear medicine are nuclear reactors, radionuclide generators, and particle accelerators (typically cyclotrons). The reactor method is the most common in the preparation of radiopharmaceuticals for scintigraphy and SPECT, but it does not allow to produce a number of important PET radioisotopes. Production of isotopes for PET is carried out in a cyclotron by “bombing” the target with accelerated charged particles.
A PET facility must include cyclotron radiochemical complex for production of radionuclides and radiopharmaceuticals, a positron emission tomography machine (with an experimental laboratory), and workstations for processing the information obtained from the PET study. Figure 10.1 shows an external view of a horizontal cyclotron and a laboratory for synthesis of radiopharmaceuticals and quality control.
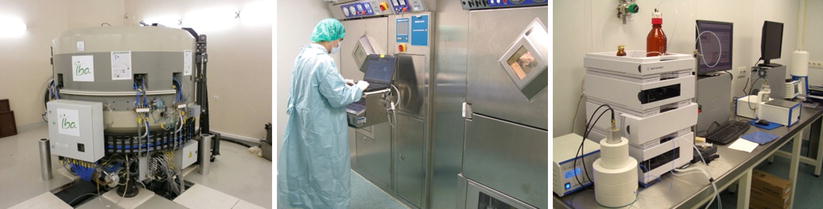
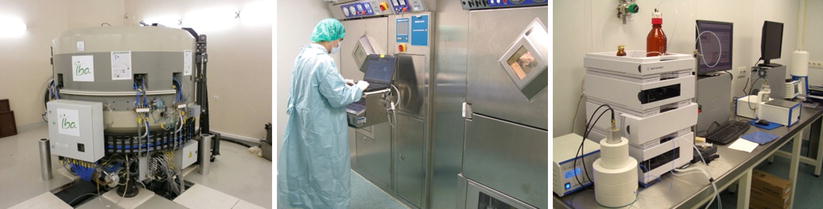
Fig. 10.1
From left to right: an open horizontal cyclotron, radiopharmaceutical synthesis laboratory, and RP quality control laboratory
The cyclotron accelerates charged particles (protons, deuterons) H/D to an energy of 18 MeV (protons)/9 MeV (deuterons), after which the accelerated particles bombard the target material. The beam energy is one of the key parameters of an accelerator, since with its increase the possibility to obtain a larger number of radionuclides occurs. For routine production of medical radionuclides, a beam energy of the order of several tens of MeV is sufficient. For producing 18F, the N.N. Blokhin Russian Cancer Research Center uses a cyclotron Cyclone 18/9 (IBA, Belgium) with a beam energy of 18 MeV for protons and 9 MeV for deuterons. Another important indicator for producing radionuclides is a beam current: the higher it is, the more intense is the production. For a mid-sized target (MV) with the volume of 1.2 ml, a working current value is 25 mA; for larger (XXL) volume of about 3.0 ml, it is as high as 80 μA. In order to prevent possible accidents, there is a safety system provided in the cyclotron, since the excess of the permissible value of the current during irradiation can cause a failure of the entire target.
Isotopes of chemical elements presented in live biological samples in large amounts are used as the target substance, water-enriched isotope 18O, nitrogen gas N2, natural water H2Or, and gas xenon 124Xe, as well as solids tellurium 124Te, thallium 203TI, zinc 68Zn, cadmium 112Cd, etc., which may substitute stable isotopes, normally available in biological molecules, without altering their physiological properties. As a result of the bombardment and nuclear reactions (p, n), radionuclides F-18, C-11, N-13, I-123, TI-201, Ga-67, and In-111 are formed and used subsequently to label radiopharmaceuticals (RP) administered to a patient in the PET study. An example of a chemical analogue may be 18F-fluorodeoxyglucose (FDG), in which hydrogen in glucose is replaced by isotope 18F.
An example of a natural substrate is water labeled with 15О (Н2 15О). Properties of chemical ligands that bind to ions of a radioactive isotope provide a high accumulation of RP in the studied organ with a minimal accumulation in other tissues. The main factors affecting the biodistribution of radiopharmaceuticals (RP) include a strong binding to blood proteins (e.g., to HSA), lipophilicity, and ionizing properties of (since lipophilic and nonionic compounds are involved in the rapid membrane transport), as well as the type of elimination (preferably excretion via the liver and kidneys).
Table 10.1 lists some of radiopharmaceuticals used in PET and presents their basic properties.
Table 10.1
Examples of the most commonly used radiopharmaceuticals and their properties
Radioisotope | Half-life | Predecessor | RP | Application |
---|---|---|---|---|
F-18 | 109.7 min | [18F]Fluoride nucleophilic substitution | [18F] FDG fluorodeoxyglucose | GLA metabolism |
[18F] FTHA fluorine-substituted fatty acids | β-oxidation | |||
[18F]-misonidazole | Hypoxia | |||
[18F] N-metilspiperon | D2 receptors | |||
16α-[18F]-fluoroestradiol | Breast cancers | |||
[18F]-fluorocholine | Prostate cancer and its metastases; brain tumors | |||
[18F]-thymidine | Proliferative activity rate | |||
[18F]F2 electrophilic substitution | 6-[18F]-fluoro-l-dopa | Dopaminergic function | ||
2-[18F]-fluoro-l–m-tyrosine | Protein synthesis, amino acid transport | |||
5-[18F]-fluorouracil | Tumor treatment control | |||
С-11 | 20.38 min | [11C] CH3I (methyl iodide) | [11C]-methionine | Protein synthesis, brain tumors |
[11C] methoxy-ephedrine | Adrenergic innervation of the heart | |||
[11C]raclopride | D2 receptors | |||
[N-methyl-11C]flumazenil | Benzodiazepine receptors | |||
[11C] СО2 | [11C]acetate | Consumption of О2 by myocardium | ||
[11C]palmitic acid | β-oxidation | |||
N-13 | 9.96 min | [13 N]NH3 | [13N]NH3 | Regulation of cardiac and cerebral blood flow |
18F-FDG has one of the longest half-life and a wide range of applications, so it is most commonly used in PET studies. In oncology, a number of radiopharmaceuticals based on 18F are used to assess various aspects of tumor metabolism.
Although radionuclide 18F produced by a cyclotron is radioactive, it exhibits the same properties as the stable isotope of fluorine (19F). The technological process of RP synthesis involves a minimum number of steps and processes to reduce the synthesis time, which in turn can significantly increase the yield of the radiopharmaceutical, given a short half-life of 18F (less than 110 min). The synthesis process is carried out in an automatic mode on the compact synthesis modules allowing to perform multistep chemical processes (Fig. 10.2). The automated synthesis modules are arranged in special airtight boxes designed to work with the activities of 15 Ci for 18F and supporting “B” class of cleanliness inside. Facilities for synthesis and packaging of radiopharmaceuticals are equipped with a special system of supply and exhaust ventilation, ensuring a pressure drop that supports the purity class “C” at the premises and gateways and class “B” in microbiological laboratories. The process of synthesis starts with the preparation of all raw materials by an engineer radiochemist. Before starting the procedure, the engineer radiochemist collects all the necessary materials and reagents in the warehouse and delivers them to the preparatory synthesis room through an active pass-through window. The preparation for synthesis involves assembling a disposable fluid processor and preliminary testing. Before this, the functional activation of disposable columns is performed, required for the separation of 18F from enriched water, conducting chemical reactions, as well as purification of synthesized radiopharmaceuticals from by-products and impurities.
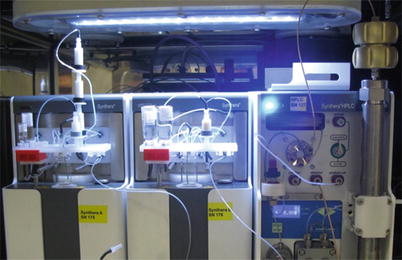
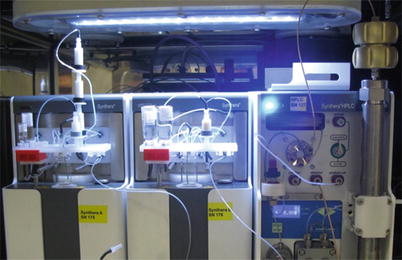
Fig. 10.2
The latest generation of synthesis modules: IBA SynthERA II (left and center), SynthERA HPLC module (right)—a HPLC system
The synthesis time depends on the type of the radiopharmaceutical synthesized and can range from 15 (for sodium fluoride) to 70 min (for fluoroethyl tyrosine). Upon completion of the synthesis, the synthesized radiopharmaceutical is automatically transmitted to the dispensing module, located in the same room. The dispensing module is an automated robotic system (Theodorico with an autoclave option). The radiopharmaceutical is dispensed into open vials in a sterile laminar airflow. Due to a multistage filtration system of the supplied air, the cleanliness class A is achieved in the chamber. The radiopharmaceutical can be dispensed both into sterile glass vials and sterile syringes. Dispensing is performed using sets of disposable sterile lines, vessels, and 220 nm filters used to filter radiopharmaceuticals. The first prefilled vial from each batch of the radiopharmaceutical in a protective container is transferred to the quality control laboratory. The vial is opened in a fume hood with a lead shielding; samples are taken in compliance with all the required procedures. RP is tested with respect to the following parameters: radiochemical purity, chemical purity, radionuclide purity, half-life, osmotic pressure and pH, endotoxin test, and sterility of the product (testing is performed by a random selection method a few times a month). After quality control, the radiopharmaceutical safety data sheet is issued. One vial is a retain sample and shall be kept for 3 years. The remaining vials with the radiopharmaceutical together with the safety data sheet that guarantees the safety of the produced batch are transferred to the procedural space of the PET unit.
During a PET study, the radiopharmaceutical is injected into the cubital vein, and the drug enters the tumor with the bloodstream, where it is selectively absorbed. Decomposing, the radiopharmaceutical emits a positron that, in a collision with an electron of the tissue (annihilation), generates two photons with an energy of 511 MeV, flying in the opposite directions. Such photons are called annihilation quanta. These photons are recorded by a multiple ring system of the PET scanner detectors. The computer calculates the coordinates of an area with a high frequency of annihilations, i.e., the areas of RP accumulation, and the reconstruction algorithm builds an isotope image of the tumor. In other words, the method is based on identification of areas with radiopharmaceutical accumulation specific for consumption of, for example, glucose. Since the metabolism of most tumors is more “aggressive” than that of normal tissue, therefore, the consumption of glucose and other metabolites is higher. Thus, images visualize lesions with increased tissue metabolism.
Performing PET with different, highly specific radiopharmaceuticals, “tropic” to certain types of tumors, provides valuable information about the state of metabolism in tumor lesions. This also allows for a semiquantitative estimate of metabolism by calculating the standard level of the radiopharmaceutical uptake (standard uptake value (SUV)), which is the ratio of the RP radioactivity in the region of interest (Bq/g) to the introduced activity of the radiopharmaceutical (Bq) per unit of patient’s body weight (Strauss and Conti 1991).
The issues of anatomical spatial visualization in radionuclide studies have been solved by the creation of a combined PET/CT system (and subsequently PET/MRI systems). The advantages of such diagnostic systems are obvious: regions of functional disorders identified by radionuclide imaging with spatial resolution of 5–7 mm are localized with the maximum accuracy based on anatomical references on the tomographic slices with the spatial resolution of 0.5 mm (Khmelev 2016). Possibilities of hybrid PET/CT or PET/MRI systems are not limited to spatial coregistration of PET and CT/MRI images, including 3D reconstruction of bone structures or the major arteries, but allow to investigate in one study, for example, hemodynamic changes in tumors using PET and CT perfusion protocols. Since 2004, mono-PET scanners have been no longer manufactured; they were replaced by the so-called hybrid PET/CT and PET/MRI systems, whose number is growing steadily worldwide. An extremely important aspect is the fact that the studies are performed in the format of a single procedure, under condition of patient stability. PET-CT is a hybrid system, where the CT scanner provides high-resolution anatomical images and a PET scanner provides functional, “molecular” images. Both scanners are used sequentially, in one diagnostic study. The result is combined (coregistred) CT and PET images. CT sections allow for a correction of the X-ray attenuation in the body tissues, needed for PET. Molecular PET images showing the spatial distribution of biochemical, metabolic activity in the body’s tissues are “tied” to the anatomical structures on CT slices. Figure 10.3 shows a PET/CT scanner with automatic devices for administration of radiopharmaceuticals and iodine-containing contrast agent, installed at the N.N. Blokhin Russian Cancer Research Center.
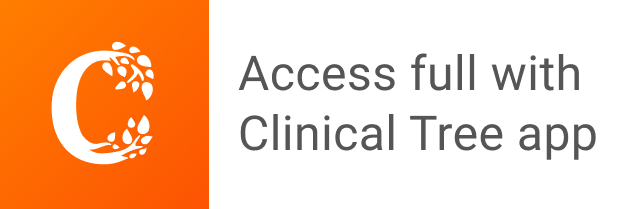