Preoperative mapping has revolutionized neurosurgical care for brain tumor patients. Maximizing resections has improved diagnosis, optimized treatment algorithms, and decreased potentially devastating postoperative deficits. Although mapping has multiple steps and complimentary localization sources, diffusion tensor imaging (DTI) excels in its essential role in depicting white matter tracts. A thorough understanding of DTI, data visualization methods, and limitations with mastery of functional and dysfunctional white matter anatomy is necessary to realize the potential of DTI. By establishing spatial relationships between lesion borders and functional networks preoperatively and intraoperatively, DTI is central to high-risk neurosurgical resections and becoming the standard of care.
Key points
- •
Preoperative mapping has revolutionized the neurosurgical care of brain tumor patients.
- •
Maximizing resections more safely has improved diagnosis, optimized treatment algorithms, and significantly decreased potentially devastating postoperative deficits associated with injury to functional brain networks.
- •
Although this mapping process has multiple steps and complimentary localization sources, diffusion tensor imaging (DTI) stands out for its essential role in depicting individual white matter tracts.
- •
A thorough understanding of DTI technique, data visualization, and limitations with a mastery of functional and dysfunctional white matter anatomy is necessary to realize the potential of this technology.
- •
By establishing spatial relationships between specific lesion borders and functional networks preoperatively and perioperatively, DTI is cementing its role in high-risk neurosurgical resections and becoming the standard of care.
Introduction
Neurosurgical care of brain tumor patients is undergoing a major shift with the emergence of preoperative brain mapping using advanced imaging techniques. This shift involves improved diagnostic and prognostic measures as well as improved patient outcomes, including significantly decreased morbidity in an otherwise inherently high-risk arena. Although preoperative mapping in tumor patients is a multistep process, it is diffusion tensor imaging (DTI) that has made the most profound impact, taking an expedited translational route from research to clinical practice. As with any tool, it is only as powerful as the person using it. Understanding the DTI technique, data visualization methods, effect of pathologic processes, and technical limitations is essential in the application to preoperative brain mapping. Combining the DTI data with expertise in white matter anatomy and functional and dysfunctional brain networks enables the physician to create a patient-specific neurosurgical plan that defines the spatial relationships between the lesion and functional brain networks, thereby guiding intraoperative assessments. The following explores the emerging and powerful clinical application of preoperative DTI.
Introduction
Neurosurgical care of brain tumor patients is undergoing a major shift with the emergence of preoperative brain mapping using advanced imaging techniques. This shift involves improved diagnostic and prognostic measures as well as improved patient outcomes, including significantly decreased morbidity in an otherwise inherently high-risk arena. Although preoperative mapping in tumor patients is a multistep process, it is diffusion tensor imaging (DTI) that has made the most profound impact, taking an expedited translational route from research to clinical practice. As with any tool, it is only as powerful as the person using it. Understanding the DTI technique, data visualization methods, effect of pathologic processes, and technical limitations is essential in the application to preoperative brain mapping. Combining the DTI data with expertise in white matter anatomy and functional and dysfunctional brain networks enables the physician to create a patient-specific neurosurgical plan that defines the spatial relationships between the lesion and functional brain networks, thereby guiding intraoperative assessments. The following explores the emerging and powerful clinical application of preoperative DTI.
Rationale for brain tumor surgery and preoperative diffusion tensor imaging
The primary goals for neurosurgical resection of brain tumors are to establish a histologic diagnosis and achieve maximal cytoreduction. Because of the histologic heterogeneity in gliomas, gross total resections are preferable to subtotal resections or biopsies for accurate diagnosis, biomarker analysis, and optimization of treatment algorithms. Treatment algorithms usually include adjuvant radiotherapy, chemotherapy, or both. Maximal cytoreduction has been theorized to decrease cell populations that could convert to higher grade neoplasms and improve the effectiveness of adjuvant therapies by altering cell kinetics and reducing cell populations resistant to chemoradiation. Point of fact, resection extent of high-grade and low-grade gliomas has been shown to correlate with improved survival. Furthermore, up to 53% of glioma patients may show improved neurologic function after resection. Operative excision of primary brain tumors can also decrease steroid dependence and seizure activity. For the foreseeable future, surgical resection of brain tumors will play a major role in brain tumor therapy.
Before the era of modern preoperative brain mapping, neurologic complication rates for brain tumor resections ranged from 7% to 26%. However, preoperative mapping has the potential to decrease postoperative complications to well below these standards. DTI shows particular promise to improve surgical outcomes in guiding intraoperative functional resection boundaries. During initial internal translation of preoperative DTI at the Medical College of Wisconsin (MCW) in 2004, a pilot study compared postoperative outcomes after resections of left dominant high-risk posterior frontal lobe tumors in 18 patients before DTI implementation and 15 patients after DTI implementation. The patient groups were matched by age, gender, histology, tumor size, and location, and with resections performed by the same neurosurgeon with identical technique. Postoperative edema and/or surgical injury resulted in speech and motor deficits in 44% and 47% of the patients in each group, respectively. However, 39% of patients without the advantage afforded by preoperative DTI had persistent speech and/or motor deficits at 1 month after surgery, resulting from surgical injury to eloquent networks. This compared with only 7% ( P <.05) for those patients with preoperative DTI available to guide resection boundaries. Recovery of deficits was presumed to be owing to resolution of postoperative edema. Since then, with further refinements in the DTI application, postoperative neurologic complication rates have fallen to less than half of this benchmark.
Other investigators utilized a prospective, randomized design to demonstrate the impact of DTI integrated tract data into neurosurgical navigational systems. In a prospective, randomized investigation, the effect of DTI on survival and postoperative neurologic outcomes was assessed. The results showed a significantly decreased incidence in postoperative motor deficits from 33% (120 patients) to 15% (118 patients) in brain tumor patients with motor tract involvement, by exporting preoperative DTI to the intraoperative navigational system. Significantly higher 6-month Karnofsky performance scores were evident in the latter group. In addition, survival was positively impacted. For the high-grade tumor subgroup (81 patients), median survival increased significantly from 14 to 21 months with the intraoperative use of preoperative DTI data. Mounting experimental data and anecdotal experience are securing a place for preoperative DTI in treatment algorithms for brain tumor patients.
Understanding white matter anatomy
A thorough understanding of white matter anatomy as reflected by DTI is required for the technique to impact surgical decision making. This requires recognition that DTI is an imperfect indicator of white matter structure and function, and that the understanding of the same in the human animal is evolving. We are some distance from fully understanding the functionality of complex human white matter networks. To date, our understanding of white matter functional networks is derived from 2 main lines of evidence, namely experimental nonhuman animal tracings and human lesion deficit localization inferences. Inferences about white matter functionality based on anatomic connectivity studies are limited by our incomplete understanding of functional cortical networks. Inferences about white matter deficits associated with surgical injury based on lesion-localization studies are limited by several factors. Most reports of deficits associated with specific white matter injury provide no patient population denominator, and as such the deficit predictability of any one individual is unknown. This may be influenced by individual variability as well as redundancy inherent in the networks. Lesions involving adjacent functional cortex or functionally distinct white matter pathways in the same anatomic region complicate lesion localization analyses further.
More elementary functional networks, such as primary motor and vision, are fairly well understood and have been deliberately avoided during neurosurgical procedures for many years. Preoperative DTI can be readily translated to complement surgical decision making in avoiding motor or vision deficits. Conversely, identifying critical white matter networks subserving higher cognitive functions is complicated by our evolving understanding of these functional networks, limitations of functional magnetic resonance imaging (fMRI), limitations of DTI, and patient variability. Autoradiographic tract tracing studies in nonhuman primates have revealed a tremendous amount of detail regarding white matter tract organization. Much of this information is directly transferable to the human brain. In other cases, however, it is not. For example, the lack of complex speech and language capabilities in nonhuman primates remains a major obstacle in applying these data directly to the human language system on a patient-by-patient basis. Emerging theories of ventral and dorsal language organization may aid in establishing which association tracts are necessary and thus to be avoided during surgery. Ultimately, our current understanding of cognitive white matter functional networks may only provide a frame of reference by which to impart preoperative risks to our neurosurgical colleagues. However, DTI as is can guide intraoperative mapping strategies. Reviews of white matter connectivity and deficits associated with lesions are available, much of which is also reflected in Tables 1 and 2 .
Tract | Reciprocal Connections | Proposed Functions | Proposed or Observed Deficits |
---|---|---|---|
SLF I | Caudal aspect of the superior parietal lobule, and superior frontal gyrus (BA 6, 9, including SMA region). | Higher-order motor function. Initiation of planned motor movements and selection of competing conditional associative motor tasks, such as driving. | Compromised selection of competing conditional associative motor tasks. |
SLF II | Caudal aspect of the inferior parietal lobule and lower bank of the intraparietal sulcus and frontal lobe (BA 6, 8, 9, 46). | Conveys visuospatial information to prefrontal cortex. Ventral component of the dorsal visual processing stream, involved in spatial attention and engagement in the environment. | Visuospatial and visual attention deficits. |
SLF III | Rostral portion of the inferior parietal lobule and parietal operculum and frontal lobe (BA 6, 44, ventral 46). | Involved in goal-direction motor behavior. Involved in imitation, and gestural and possible linguistic communication. Likely involved in phonemic and articulatory aspects of language, as the dorsal language pathway of the dominant hemisphere. | Ideomotor apraxia ( left ). Phonemic and articulatory deficits ( left ). |
SLF IV | Caudal aspect of the superior temporal lobe, including superior temporal gyrus and sulcus, and frontal lobe (dorsal BA 8, 46, 6). | Auditory spatial bundle. May not be directly linked to language areas as previously thought. | Deficits in auditory discrimination. |
SFOF | Medial preoccipital area, lateral-dorsal preoccipital area, medial parietal area, caudal cingulate gyrus, caudal inferior parietal lobule, and dorsolateral prefrontal cortex. Ventrally rests on the SCF. | Dorsal component of the dorsal visual processing stream. Involved in action tracking and reaching, visual guidance of movements, maintenance of vigilance and spatial aspects of attention through connections to supplementary eye fields. | Deficits in visuospatial processing. Optic ataxia. |
IFOF | Presence is disputed, but believed by advocates to connect occipital and posterior temporal lobes, and dorsal/ventral prefrontal cortex. Posterior terminations include posterior MTG and ITG, fusiform/lingual gyri. | Conveys information between visual association areas and frontal lobes. Implicated in ventral (semantic) language network functions, based on intraoperative stimulation studies. | Semantic paraphasias with intraoperative stimulation. Possible visuospatial and visual recognition deficits. |
ILF | Ventral occipital lobe, parietal lobe including intraparietal sulcus, and temporal lobe including parahippocampal gyrus. | Component of ventral processing stream that is involved in object/place/face/word (left) recognition. Involved in visual associative memory, analysis of visual motion, visual spatial analysis and attention. Plays subsidiary role in the ventral language stream. | Visual agnosias (objects, words, faces, places). Visual memory deficits. Visual hypo-emotionality. |
UF | Anterior and medial temporal lobe (rostral STG and ITG, parahippocampal gyrus, amygdala), and orbitofrontal/medial prefrontal/ventrolateral prefrontal cortex. Also amygdala to anterior and inferior temporal cortex. | Connects areas of sound recognition, memory recognition, and is involved in processing of emotion, inhibition and self-regulation. Important in the interactions between auditory stimuli, cognition and emotion. Right: Engaging visual or multimodal imagery necessary for retrieval of autobiographical or event memories. Left: Storage and access to remote semantic and lexical memories. | Right : Disturbances in retrieval of episodic, context-dependent memory (memory of personal experiences). Left : Semantic, context-free memory (learned concepts and facts). |
CG | Limbic pathway connecting septal area to the uncus, coursing within the cingulate and parahippocampal gyri. Anterior CG: Connects frontal lobe, parietal lobe, amygdala, hippocampus, medial dorsal thalamus, basal ganglia, brainstem motor nuclei, and hypothalamus. Posterior CG: Connects temporal association, medial temporal, parietal, orbitofrontal, and dorsolateral prefrontal cortex. | Anterior CG : Attention, volitional control of cognition and motor functions. Anterior CG (rostral): Involved in emotion and visceral functions. Anterior CG (caudal): Involved in cognition and higher-order motor control. Posterior CG: Monitoring sensory events and behavior in the service of memory and spatial orientation. Visuospatial processing and verbal memory. Processing of phasic pain and transforming aversive input into movements. Possible role in stimulus-driven, smooth pursuit, and reflexive eye movements. | Anterior CG : Pain unconsciousness. Akinetic mutism ( left ). Deficits of saccades and internally driven eye movements. Posterior CG : Retrosplenial amnesia. Visuospatial, visual memory and verbal memory deficits. |
Tract | Projections and Connections | Observed Deficits |
---|---|---|
SCF | Corticosubcortical connections from anterior cingulate gyrus and pre-SMA/SMA region to the corpus striatum. | Left : Akinetic mutism. Disorders of preparation and initiation of speech. Transcortical motor aphasia, anomia, and delayed initiation of speech with preserved articulation observed with intraoperative stimulation. |
ALIC | Corticofugal motor and thalamocortical sensory projection fibers form compact WM bundles in the corona radiata and internal capsule. The ALIC contains anterior thalamic sensory and FPT motor fibers. | Impairment of inhibitory reflexive eye saccades with injury to the FPT connecting the dorsolateral PFC to superior colliculi. Ipsilesional conjugate eye deviation with injury to the FPT connected to the superior colliculus or paramedian pontine reticular formation. |
GIC | Corticofugal motor and thalamocortical sensory projection fibers make up compact WM bundles in the corona radiata and internal capsule. The GIC contains anterior and inferior thalamic fibers and the corticobulbar tract. | GIC: Contralateral faciolingual weakness. Subcortical dementia and executive memory disorder with injuries to thalamofrontal/thalamolimbic fibers. Contralesional intermittent failure to maintain sustained muscle contraction. GIC and ALIC: Impaired motor control circuit with injury to vestibulocerebellar and cerebello-rubro-thalamic pathways and fibers from SMA and prefrontal region projecting to the thalamus. GIC–PLIC junction: Contralesional motor and sensory deficits of fingers, face, and tongue. Verbal memory deterioration (left dominant). Dizziness/vertigo with injury to the vestibulothalamic pathway (right or left). |
PLIC | Corticofugal motor and thalamocortical sensory projection fibers make up compact WM bundles in the corona radiata and internal capsule. The PLIC contains superior, posterior, and inferolateral thalamic fibers, the corticospinal tract, and the TPOPT. The retrolenticuar portion of the PLIC contains components of the optic and acoustic radiations. | Apathy and impaired consciousness. Contralesional hemiparesis/hemiplegia. Contralesional hemianesthesia with injury to the thalamocortical sensory pathway. Contralesional hemiataxia with injury to cerebellar dentato-rubro-thalamocortical and corticopontocerebellar pathways. Verbal memory loss ( left ). Ipsilesional conjugate eye deviation with injury to the TPOPT connected to the superior colliculus or paramedian pontine reticular formation. Impairment in the reflexive eye saccades owing to the injury to the parietotectal fibers. |
FX | Limbic structure connecting the hippocampus with the hypothalamus and basal forebrain nuclei. | Memory deficits, learning dysfunction, antegrade amnesia, temporally graded retrograde amnesia. Visual memory deficits ( right ). Verbal memory deficits ( left ). |
OR | Project from the lateral geniculate nucleus to the primary visual cortex. Superior fibers course backward toward the occipital lobe, and inferior fibers course anteroinferiorly around the temporal horn before coursing posteriorly to the primary visual cortex and form Meyer’s loop. | Contralesional visual field deficits. Lower visual field deficit from injury to superior fibers. Superior visual field deficit from injury to inferior fibers. Visual field deficits may occur from injury to the optic radiation located in the retrolenticular portion of the PLIC. |
AR | Project from medial geniculate nucleus to the primary auditory cortex. AR fibers course through the retrolenticular portion of the PLIC. | Auditory agnosia. Word deafness (bilateral or left). Amusia (right > left). Environmental sound agnosia (bilateral or right). |
CC | Connects homologous neocortical areas across hemispheres. | Genu and anterior body: Nondominant limb apraxia, frontocallosal alien hand syndrome, cognitive dysfunction. Posterior body and splenium: Left ideomotor apraxia, left hand tactile anomia, left visual field color anomia, associative visual agnosia, left visual field dyslexia, left hand agraphia, right hand constructural apraxia, left hemispatial neglect, left motor neglect, callosal alien hand syndrome, visuomotor delay, optic ataxia, and auditory disconnection. |
Functional localization sources
Given the limitations of our understanding of white matter networks and the limitations of the DTI technique, complimentary preoperative and perioperative localization sources are necessary to establish functional network proximity risks. These sources include clinical presentation, functional anatomy at standard imaging, preoperative functional mapping techniques, intraoperative functional white matter testing, and intraoperative electrocortical mapping. Each of these sources is an imperfect indicator of proximity risk alone, but together can provide critical functional information.
Clinical presentation is a localizing parameter in and of itself. A lesion-induced persistent deficit is an indicator of proximity and operative risk for that particular function. An understanding of localized lesion-induced deficits helps to determine the source of such deficits, and relevant reviews on the topic are available. A presenting deficit that persists has a fairly high positive predictive value that the lesion has proximity to eloquent structures. However, a transient deficit associated with seizure activity lowers positive predictive value considerably, owing to the potential for propagation of epileptogenic activity. A high negative predictive value (NPV) should not be assumed because seizures and persistent deficits may be absent even though a lesion has proximity to eloquent structures. Handedness determines the likelihood of left hemisphere language dominance. Right-handed individuals have a 98% chance of being left hemisphere dominant for language function. This means for every 100 mapping cases performed on right-handers, 2 of them will have significant language function in the right hemisphere. Left-handed individuals have a 67% chance of being left dominant. The remaining 33% of left handers are either right hemisphere dominant for language or have shared hemisphere function. Hemispheric dominance established by fMRI informs significance to potential language networks in the lesional hemisphere established at DTI.
Knowledge of functional anatomy at standard imaging is critical in the interpretation of preoperative DTI data. Alone, DTI can be limited in establishing functional network proximity because of pathophysiologic constraints. In clinical practice, the color-coded fractional anisotropy (CC-FA) maps are superimposed on anatomic imaging sequences to optimally characterize relationships of white matter structures to pathologic processes ( Fig. 1 ). Anatomic distortion of perilesional white matter from edema and infiltrating tumor may obscure or reorient tracts, making them difficult to discern on CC DTI. With the use of standard imaging, expected relationships of tracts to each other and to gyral and sulcal landmarks can help to predict functional network proximity when tract directions are altered or FA is reduced.
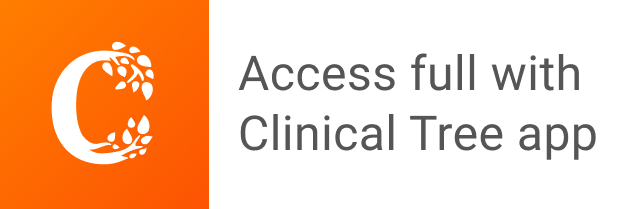