Abstract
There is growing controversy in the field of neonatal hemodynamics about the diagnosis and treatment of neonatal cardiovascular compromise in the very preterm infant. Accordingly, fundamental questions such as the timely recognition of the subpopulation of neonates at high risk for the development of hemodynamic compromise in the transitional period, the definition of individual patient-dependent blood pressure (BP) thresholds associated with inadequate tissue oxygen delivery (DO 2 ), the role of physiologic cord clamping, the recognition of the hemodynamic antecedents of peri-intraventricular hemorrhage, and other related issues are yet to be answered. In the absence of such information, applying the principles of developmental cardiovascular physiology and pathophysiology can help in establishing the diagnosis and determining the optimal management of neonatal circulatory compromise.
The past two decades have seen a growing controversy in the field of neonatal hemodynamics in general and over the diagnosis and treatment of neonatal cardiovascular insufficiency especially in the very preterm infant. The complexities and difficulties associated with the design and execution of randomized controlled trials (RCTs) targeting clinically relevant outcome measures in very preterm neonates with cardiovascular compromise are the primary reasons for the ongoing controversy. Indeed, questions such as the BP threshold associated with inadequate tissue DO 2 and thus necessitating treatment in a selected patient population can be answered only by properly designed RCTs. In the absence of such information, applying the principles of developmental cardiovascular physiology and pathophysiology can aid the diagnosis and management of neonatal circulatory compromise. In addition, designing RCTs in this area also requires a thorough understanding of developmental cardiovascular physiology.
Therefore this chapter first reviews the principles of fetal, transitional, and posttransitional hemodynamics, with an emphasis on the principles of developmental cardiovascular physiology. Building on the physiologic principles reviewed, the second part of the chapter then discusses the etiology and pathophysiology of neonatal cardiovascular compromise. The major goals of this chapter are to help the reader to appreciate the impact of immaturity and/or pathologic events on the physiology of neonatal cardiovascular transition and understand the primary factors leading to cardiovascular compromise in the preterm and term neonate. Using this knowledge, along with carefully selected and relevant information from clinical trials, the clinician can best assess and manage hemodynamic disturbance in the immediate transitional period and beyond and potentially reduce the end-organ damage caused by decreased DO 2 to the organs, especially the immature brain in affected neonates.
Keywords
blood pressure, cardiac output, developmental cardiovascular physiology, fetal circulation, hemodynamics, neonatal shock, neonate, systemic vascular resistance, transitional circulation
- •
A successful hemodynamic transition from fetal to extrauterine life is a complex process that requires the interdependent sequential physiologic changes to take place in a timely manner.
- •
Immaturity- or pathophysiology-driven disturbances of the transitional process may have significant short- and long-term consequences.
- •
Timely diagnosis and pathophysiology-targeted management of neonatal shock pose difficult challenges for the clinician.
- •
Many questions remain unanswered, including, but not restricted to, the timely recognition of the subpopulation of neonates at high risk for the development of hemodynamic compromise in the transitional period, the definition of the individual patient-dependent blood pressure thresholds associated with inadequate tissue oxygen delivery, the role of physiologic cord clamping, and the recognition of the hemodynamic antecedents of peri-intraventricular hemorrhage.
Principles of Developmental Physiology
Fetal Circulation
The fetal circulation is characterized by low systemic vascular resistance (SVR) with high systemic blood flow and high pulmonary vascular resistance with low pulmonary blood flow. Given the low oxygen tension of the fetus, the fetal circulation allows for preferential flow of the most oxygenated blood to the heart and brain, two of the three “vital organs.” With the placenta rather than the lungs being the organ of gas exchange, most of the right ventricular output is diverted through the patent ductus arteriosus (PDA) to the systemic circulation. In fact, the pulmonary blood flow constitutes only approximately 7% to 8% of the combined cardiac output in fetal lambs. However, Doppler and magnetic resonance imaging studies have shown that the proportion of combined cardiac output that supplies the lungs is significantly higher in the human fetus (11% to 25%), with some studies reporting an increase in this proportion with advancing gestational age to a peak approximately 30 weeks’ gestation. In fetal life, both ventricles contribute to the systemic blood flow, and the circulation therefore depends on the persistence of shunts via the foramen ovale and PDA between the systemic and pulmonary circuits, with the two circulations functioning in “parallel.” The right ventricle is the dominant pumping chamber, and its contribution to the combined cardiac output is approximately 60%. The combined cardiac output is in the range of 400 to 450 mL/kg/min in the fetus, which is much higher than the systemic flow after birth (approximately 200 mL/kg/min). Approximately, one-third of the combined cardiac output (150 mL/kg/min) perfuses the placenta via the umbilical vessels. However, placental blood flow decreases to 21% of the combined cardiac output near term. The umbilical vein carries the oxygenated blood from the placenta through the portal veins and the ductus venosus to the inferior vena cava (IVC) and eventually to the heart. Approximately 50% of oxygenated blood in the umbilical vein is shunted through the ductus venosus and IVC to the right atrium, where the oxygenated blood is preferentially directed to the left atrium through the patent foramen ovale. This percentage decreases as gestation advances. One of the unique characteristics of the fetal circulation is that arterial oxygen saturation (SaO 2 ) is different between the upper and lower body. Having the most oxygenated blood in the left atrium ensures supply of adequate oxygen to the heart and brain. Furthermore, in response to hypoxemia, most of the blood flow in the umbilical vein bypasses the portal circulation via the ductus venosus and again delivers the most oxygenated blood to the heart and brain.
Transitional Physiology
Transition from the fetal to the postnatal type of circulation is a complex process. In the past few years, research interest has again focused on cardiovascular and pulmonary adaptation at birth. Among others, animal and human studies have investigated the impact of the timing of cord clamping on cardiovascular and pulmonary transitional physiology. The findings of these studies have highlighted the importance of allowing for placental transfusion to take place and suggested that lung aeration should be established prior to umbilical cord clamping to ensure that the source of the left ventricular preload gradually changes over from the placenta to the lungs. The maintenance of appropriate left ventricular preload during the immediate hemodynamic transition from the fetal to the postnatal circulation has been shown to attenuate the abrupt decrease in preload and systemic blood flow seen with the practice of immediate cord clamping and is associated with improved postnatal hemodynamic stability and clinical outcomes (see Chapter 4 , Chapter 6 ). Among the clinical outcomes, improved postnatal transition, decreased need for blood transfusion, and lower incidence of intraventricular hemorrhage have been documented without significant untoward effects associated with delayed cord clamping in preterm infants. In addition, a decreased need for blood transfusion has been observed in term neonates, albeit with higher rates of jaundice and polycythemia reported. As discussed in Chapter 5 in detail, these findings have led to a departure from the traditional approach of immediate cord clamping and a move to delayed clamping of the cord for all newborns who are vigorous at birth in the absence of conditions preventing placental transfusion. Interestingly, cord milking seems to confer similar hemodynamic benefits to delayed cord clamping. However, further studies are needed to provide convincing evidence that the two procedures indeed confer the same clinical benefits and are safe for the preterm infant.
After birth, the circulation changes from parallel to series, and thus the left and right ventricular outputs must become equal. However, this process, especially in very preterm infants, is not complete for days or even weeks after birth, due to the inability of the fetal channels to close in a timely manner. The persistence of the PDA significantly alters the hemodynamics during transition and beyond and has been associated with severe and even refractory hypotension. The impact of the PDA on pulmonary and systemic blood flow in the preterm infant is discussed in Chapter 22 . At birth, the removal of the low-resistance placental circulation and the surge in catecholamines and other hormones increases the SVR. On the other hand, the pulmonary vascular resistance drops precipitously due to the act of breathing air and exposure of the pulmonary arteries to higher partial pressure of oxygen as compared with the very low level in utero. Organ blood flow also changes significantly. In the newborn lamb, cerebral blood flow (CBF) drops in response to oxygen exposure. A drop in CBF in the first few minutes after birth in normal term neonates was also reported. This drop in CBF appears to be related, at least in part, to cerebral vasoconstriction in response to the increase in arterial blood oxygen content immediately after birth. In addition, the correlation between left-to-right PDA shunting and middle cerebral artery (MCA) flow velocity (a surrogate for CBF) suggests a possible role of the ductus arteriosus in the observed reduction in CBF. Finally, especially in some very preterm neonates, the inability of the immature myocardium to pump against the suddenly increased SVR might lead to a transient decrease in systemic blood flow, which in turn could also contribute to the decrease in CBF (see Chapter 2 , Chapter 11 ).
Postnatal Circulation
Pressure, Flow, and Resistance
Poiseuille’s equation ( Q = (Δ P × π r 4 )/8 μL ) describes the factors that determine the movement of fluid through a tube. This equation helps us to understand how changes in cardiovascular parameters affect blood flow. Basically, flow (Q) is directly related to the pressure difference (Δ P ) across the vessel and the fourth power of the radius (r) and inversely related to the length (L) of the vessel and the viscosity of the fluid (μ) . Therefore blood pressure (BP) is the driving force behind moving blood through the vasculature. Because there are several differences between laminar flow of water through a tube and blood flow through the body, the relationship between the previously mentioned factors in the body does not exactly follow the equation. In addition, because we do not measure all components of this equation, in clinical practice the interaction among BP, flow, and SVR is described by using an analogy of Ohm’s law (cardiac output = pressure gradient/SVR). Therefore blood flow is directly related to BP and inversely related to SVR. Regulation of and changes in cardiac output and SVR determine the BP. In other words, systemic BP is the dependent variable of the interaction between the two independent variables: cardiac output (flow) and SVR. Of note is that, because cardiac output is also partly affected by SVR, in theory, cardiac output cannot be considered a completely independent variable.
Cardiac output is determined by heart rate, preload, myocardial contractility, and afterload. Preload can be described in terms of pressure or volume (i.e., central venous pressure or end-diastolic ventricular volume). Therefore preload is affected not only by the effective circulating blood volume but also by many other factors, such as myocardial relaxation and compliance, contractility, and afterload. The limited data available on diastolic function in the newborn in general and in preterm infants in particular suggest lower myocardial compliance and relaxation function. On the other hand, baseline myocardial contractility is high or comparable to older children, whereas the myocardial capacity to maintain contractility in the face of an increase in the afterload might be limited (see later). Afterload and SVR are related and usually change in the same direction. Yet, these two parameters are different and should not be used interchangeably. SVR is determined by the resistance of the vascular system and is regulated by changes in the diameter of the small resistance vessels, primarily the arterioles. In contrast, afterload is the force that the myocardium has to overcome to pump blood out of the ventricles during the ejection period. Wall tension can be used as a measure of afterload. Therefore, based on Laplace’s law, left ventricular afterload is directly related to the intraventricular pressure and the left ventricular diameter at the end of systole and indirectly related to the myocardial wall thickness. Indeed, changes in SVR exert their effect on afterload indirectly by affecting BP.
Organ Blood Flow Distribution
Under resting physiologic conditions, blood flow to each organ is regulated by a baseline vascular tone under the influence of the autonomic nervous system. Changes in the baseline vascular tone regulate organ blood flow. Vascular tone is regulated by local tissue (e.g., H + , CO 2 , and O 2 ), paracrine (e.g., nitric oxide [NO], prostacyclin, and endothelin-1), and neurohormonal factors, as well as by the myogenic properties of the blood vessel. Under pathologic conditions such as hypoxia-ischemia, the relative organ distribution of cardiac output favors the “vital” organs (the brain, heart, and adrenal glands). In principle, vital organ designation is operational even in fetal life. However, the vascular bed of the forebrain (cortex) might only achieve the characteristic “vital organ” vasodilatory response to a decrease in perfusion pressure late in the second trimester (see further discussion later).
Microcirculatory Physiology (see Chapter 19 )
Other than being the site of exchange of oxygen and nutrients and removal of metabolic byproducts, the microcirculation also plays a significant role in regulating systemic and local hemodynamics. The small arteries and arterioles are the main regulators of peripheral vascular resistance, and the venules and small veins play an important role as capacitance vessels. Coupling of oxygen supply and demand is one of the primary functions of the microcirculation. Oxygen delivery (DO 2 ) depends on blood flow and oxygen content. The total oxygen content of the blood (hemoglobin bound and dissolved) can be calculated based on the hemoglobin (Hb) concentration (g/dL), SaO 2 , and partial pressure of oxygen (PaO 2 ; mm Hg) in the arterial blood ([1.36 × Hb × SaO 2 ] + [0.003 × PaO 2 ]). Tissue blood flow is adjusted based on the oxygen consumption (VO 2 ) determined by the metabolic requirements. When the blood flow cannot be increased beyond a certain point, oxygen extraction is increased to meet the demand for VO 2 . Therefore VO 2 is not affected by decreases in blood flow until the tissue’s capacity to extract more oxygen is exhausted. At this point, VO 2 becomes directly flow dependent.
In healthy term infants, localized peripheral (buccal) perfusion assessed by capillary-weighted saturation using visible light spectroscopy has only a weak correlation with central blood flow during the transitional period. Therefore it is possible that under physiologic conditions, peripheral blood flow is not affected by the variability in the systemic blood flow. In other words, blood flow (cardiac output) is regulated to meet VO 2 . In ventilated preterm infants, limb blood flow assessed by near-infrared spectroscopy showed no correlation with BP. Along with the poor correlation of buccal SaO 2 with cardiac output in healthy term neonates, these findings suggest that regulation of the microcirculation and peripheral blood flow in relatively hemodynamically stable preterm and term neonates might be, at least to a certain point, independent from systemic blood flow.
Skin microcirculation has been more extensively studied in neonates. Orthogonal polarization spectral imaging studies of skin demonstrated that functional small vessel density, a measure of tissue perfusion and microcirculation, changes over the first postnatal month and directly correlates with hemoglobin concentration and environmental temperature in preterm infants. In this study, functional small vessel density was also inversely related to BP. This finding indicates that evaluation of skin microcirculation may be useful in the indirect assessment of SVR. Findings of laser Doppler flowmetry studies of the skin indicate that the relationship between peripheral microvascular blood flow and cardiovascular function evolves during the first few postnatal days and that it depends on the gestational age and sex of the patient. Indeed, the inverse relationship between microvascular blood flow and calculated SVR and mean BP immediately after delivery is no longer present by the fifth day of postnatal life. Interestingly, male preterm infants are more likely to have peripheral vasodilation at 24 hours after birth, and this may be related to the different maturational pattern of the autonomic nervous system in male compared with female neonates. A study found a higher sympathetic output at 6 hours after birth in male preterm infants compared with females; however, sympathetic tone tends to decrease in males and increase in females over time during the first 3 postnatal days. Gestational age has an inverse relationship with microvascular blood flow during the first few postnatal days. Among the most immature neonates (≤28 weeks), those who died during the transitional period had higher baseline microvascular blood flow (i.e., lower peripheral vascular resistance). These findings suggest that developmental changes in the microcirculation also play a significant role in the regulation of transitional hemodynamics, and that microcirculatory maladaptation is associated with and/or may increase the risk of mortality.
Given the limited data available and inconsistency of the findings, further studies of the regulation of microcirculation are needed to improve our understanding of the physiology and pathophysiology of the microcirculation during development and postnatal transition and to better characterize its role in the regulation of systemic hemodynamics in the preterm and term neonate.
Myocardial Function—Developmental Aspects
There are significant differences in myocardial structure and function between the immature myocardium of the neonate compared with that of the older child and adult. The immature myocardium of the preterm and term neonate has less contractile elements, higher water content, greater surface to volume ratio, and an underdeveloped sarcoplasmic reticulum. The immature myocardium primary relies on the function of L-type calcium channels and thus on extracellular calcium concentration for calcium supply necessary for muscle contraction. In children and adults, these channels serve only to trigger the release of calcium from its abundant intracellular sources in the sarcoplasmic reticulum. These characteristics of the immature myocardium explain the observed differences in myocardial compliance and contractility between preterm and term neonates and children and adults.
Echocardiographic studies have shown that the immature myocardium has a higher baseline contractile state and that contractility rapidly decreases in the face of an increase in afterload. The sensitivity of the immature myocardium to afterload means that for the same degree of rise in the afterload, the myocardium of the neonate has a more significant reduction in contractility compared with children or adults. With the rise in SVR after birth, left ventricular afterload increases. This, in turn, may lead to a significant decrease in myocardial contractility with possible clinical implications (see discussion under Myocardial Dysfunction).
Impact of the Immature Autonomic Nervous System on Regulating Cardiac Function and Vascular Tone
Circulatory function is mediated at the central and local levels through neural, hormonal, and metabolic mechanisms and reflex pathways ( Fig. 1.1 ). Integral to the regulation of cardiac function and vascular tone is the central nervous system. The medulla generates complex patterns of sympathetic, parasympathetic, and cardiovascular responses that are essential for homeostasis, as well as behavioral patterning of autonomic activity. The balance between sympathetic and parasympathetic outflow to the heart and blood vessels is regulated by peripheral baroreceptors and chemoreceptors in the aortic arch and carotid sinus, as well as by the mechanoreceptors in the heart and lungs. Although many of these pathways have been identified, much work remains to delineate the adaptation of cardiovascular control in the immature infant where the maturation of the many components of this complex system is at varying pace and has been shown to lead to instability in autonomic function and maintenance of adequate organ blood flow and BP. The effect of the dynamic nature of the developing system on cardiovascular function is unclear, but it may have short- and long-term implications for neonates born premature or with growth restriction.
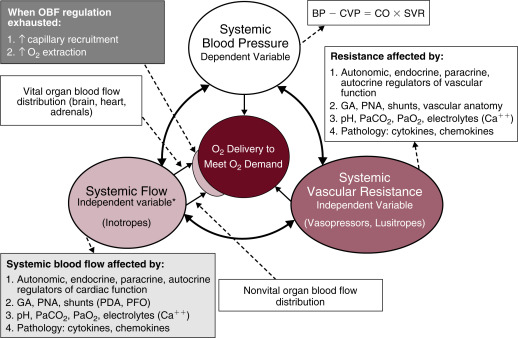
Heart rate variability analysis is a noninvasive tool used to assess the sympathetic and parasympathetic modulation of the cardiovascular system over a relatively short period of time. This method has been found to be useful in conditions in which cardiac output has been impacted, such as in patients with sepsis (see Chapter 20 ). Heart rate variability analysis holds promise to further characterize the autonomic control of cardiovascular function because the relationship among heart rate variability, sympathovagal balance, and the modulation of the renin-angiotensin-aldosterone system in various pathophysiologic states can be explored.
Developmental Cardiovascular Pathophysiology: Etiology and Pathophysiology of Neonatal Shock
To ensure normal cellular function and maintenance of structural integrity, delivery of oxygen must meet cellular oxygen demand. DO 2 is determined by the oxygen content of the blood and cardiac output (see earlier). However, cardiac output can deliver oxygen effectively only to the organs if perfusion pressure (BP) is maintained in a range appropriate to the given conditions of the cardiovascular system. Because BP is determined by the interaction between SVR and cardiac output (BP = SVR × systemic blood flow; see Fig. 1.1 ), the complex interdependence between perfusion pressure and systemic blood flow mandates that, if possible, both should be monitored in critically ill neonates (see Chapter 21 ).
Indeed, if SVR is too low, BP (perfusion pressure) may drop below a critical level at which cellular DO 2 becomes compromised despite normal or even high cardiac output. However, if SVR is too high, cardiac output and thus organ perfusion may decrease to a critical level so that cellular DO 2 becomes compromised despite maintenance of BP in the perceived normal range. Therefore the use of either the BP or cardiac output alone for the assessment of the cardiovascular status is misleading, especially under certain critical circumstances in preterm and term neonates. Unfortunately, although BP can be continuously monitored, there are only few, recently developed, and not yet fully validated invasive and noninvasive bedside techniques we can use to continuously monitor systemic perfusion, in absolute numbers in the critically ill neonate (see Chapter 21 ). Therefore in most intensive care units the clinician has been left with monitoring the indirect and rather insensitive and nonspecific measures of organ perfusion, such as urine output and capillary refill time (CRT) (see Chapter 26 ). Monitoring the time course of the development and cessation of lactic acidosis is the most specific indirect measure of the status of tissue perfusion, and it has become available from small blood samples, along with routine blood-gas analysis. However, this measure also has its limitations because elevated serum lactic acid levels may represent an ongoing impairment in tissue oxygenation or a previous event with improvement in tissue perfusion (“washout phenomenon”). Thus serum lactic acid concentration needs to be sequentially monitored and a single value may not provide appropriate information regarding tissue perfusion. Furthermore, when epinephrine is being administered, epinephrine-induced specific increases in lactic acid levels occur independent of the state of tissue perfusion.
Because it is a common practice to routinely measure BP in neonates, population-based normative data are available for the statistically defined normal ranges of BP in preterm and term neonates. It is very likely that the 5th or 10th percentiles of these gestational- and postnatal-age dependent normative data used to define hypotension do not represent BP values in every patient where autoregulation of organ blood flow or organ blood flow itself is necessarily compromised. Although findings have described the possible lower limits of BP below which autoregulation of CBF, cerebral function, and, finally, cerebral perfusion are impaired in very low birth weight (VLBW) preterm infants ( Fig. 1.2 ), the true impact of gestational and postnatal age, the individual patient’s ability to compensate with increased cardiac output and appropriate regulation of organ blood flow, and the underlying pathophysiology on the dependency of CBF on BP in this population remain to be determined. It is particularly interesting that two studies using different methods and patients with different gestational and postnatal age have found that the lower elbow of the CBF autoregulatory curve might be at 29 mm Hg of mean BP. Yet, there is not sufficient evidence to recommend that mean BP in preterm neonates during the first postnatal days be kept greater than the breakpoint. Several epidemiologic studies have demonstrated that hypotension and/or low systemic perfusion are associated with increased mortality and morbidity in the neonatal patient population. Other studies found an increase in mortality and morbidity in preterm infants who received treatment for hypotension. Due to the retrospective and uncontrolled nature of these studies, it is hard to tease out the cause of adverse outcome associated with hypotension. It is possible that the poor outcome associated with hypotension is multifactorial, and it may be due to the direct effect of hypotension on organ perfusion; the inappropriate use and titration of vasopressor-inotropes, inotropes, or lusitropes; coexistence of other pathologies with hypotension as a marker of disease severity; or a combination of all of these factors.
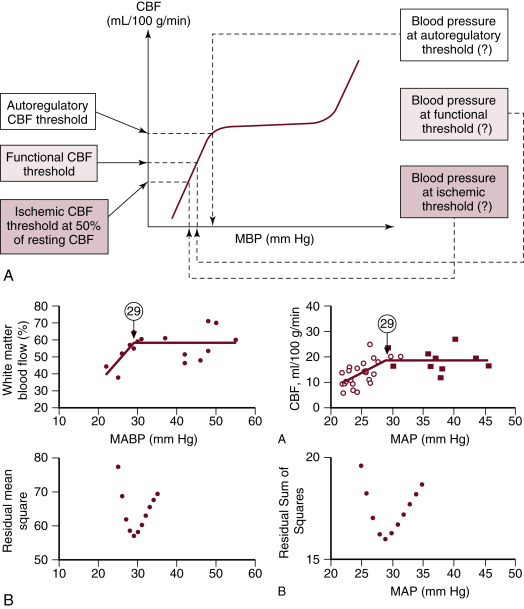
Definition and Phases of Shock
Shock is defined as a condition in which supply of oxygen to the tissues does not meet oxygen demand. In the initial “compensated” phase of shock, neuroendocrine compensatory mechanisms and increased tissue oxygen extraction maintain perfusion pressure, blood flow, and oxygen supply to the vital organs (heart, brain, and adrenal glands) at the expense of blood flow to the rest of the body. This is achieved by selective vasoconstriction of the resistance vessels in the nonvital organs leading to maintenance of BP in the normal range and redistribution of blood flow to the vital organs. Low normal to normal BP, increased heart rate, cold extremities, delayed CRT, and oliguria are the hallmarks of this phase. Unfortunately, although these clinical signs are useful in detecting early shock in pediatric and adult patients, they are of limited value in neonates, especially in preterm infants in the immediate postnatal period. Indeed, in preterm infants immediately after birth, shock is rarely diagnosed in this phase, and it is usually only recognized in the second, “uncompensated,” phase. In the uncompensated phase of shock, the neuroendocrine compensatory mechanisms fail and hypotension, decreased vital and nonvital organ perfusion, and DO 2 develop. These events first result in the loss of vital organ blood flow autoregulation and the development of lactic acidosis, and, if the process progresses, cellular function and then structural integrity become compromised. However, even in the compensated phase, recognition of shock may be delayed because of the uncertainty about the definition of hypotension in preterm infants. Finally, if treatment is delayed or ineffective, shock progresses to its final “irreversible” phase . In this phase, irreparable cellular damage occurs in all organs and therapeutic interventions will fail to sustain life.
Etiology of Neonatal Shock
Neonatal shock may develop because of volume loss (absolute hypovolemia), myocardial dysfunction, abnormal peripheral vasoregulation, or a combination of two or all of these factors.
Hypovolemia
Adequate preload is essential for maintaining normal cardiac output and organ blood flow. Therefore pathologic conditions associated with absolute or relative hypovolemia can lead to a decrease in cardiac output, poor tissue perfusion, and shock. Although absolute hypovolemia is a common cause of shock in the pediatric population, in neonates in the immediate postnatal period it is rarely the primary cause. Neonates are born with approximately 80 to 100 mL/kg of blood volume, and only a significant drop in blood volume leads to hypotension. Perinatal events that can cause hypovolemia include a tight nuchal cord, cord avulsion, cord prolapse, placental abruption, fetomaternal transfusion, and birth trauma such as subgaleal hemorrhage. Fortunately, these perinatal events either do not result in significant hypovolemia and shock in most instances (e.g., placental abruption) or their occurrence is very rare (e.g., cord avulsion). Another cause of absolute hypovolemia is transepidermal water loss in extremely low birth weight (ELBW) infants in the immediate postnatal period. The potential role of early umbilical cord clamping in relative hypovolemia has recently been raised.
To explore the role of intravascular volume status in the occurrence of hypotension, several investigators have evaluated the relationship between blood volume and systemic arterial BP in normotensive and hypotensive preterm infants. Bauer et al. measured blood volume in 43 preterm neonates during the first 2 postnatal days and found a weak but statistically significant positive correlation between BP and blood volume. However, there was no correlation between blood volume and BP until blood volume exceeded 100 mL/kg. Barr et al. found no relationship between arterial mean BP and blood volume in preterm infants and no difference in blood volume between hypotensive and normotensive infants. Similarly, Wright and Goodall reported no relationship between blood volume and BP in preterm neonates in the immediate postnatal period. Therefore absolute hypovolemia was for a long period thought to be an unlikely primary cause of hypotension in preterm infants in the immediate postnatal period. This notion was further supported by the fact that dopamine was shown to be more effective than volume administration in improving BP in preterm infants during the first days after delivery.
On the other hand, delayed cord clamping and cord milking have been shown to confer short-term hemodynamic benefits, and, in addition to the improved maintenance of left ventricular preload during early hemodynamic transition, these beneficial hemodynamic effects are also thought to be the result of the associated increases in blood volume. In summary, it is unclear at present to what extent and in which patients the hypovolemic component is a factor during hemodynamic transition.
Myocardial Dysfunction (see Chapter 26 )
As discussed earlier, there are considerable differences in the structure and function of the myocardium among preterm and term infants and children. The significant immaturity of the myocardium of the preterm infant explains, at least in part, why these patients are prone to develop myocardial failure following delivery.
The limited capacity to increase contractility greater than the baseline makes the immature myocardium prone to fail when SVR abruptly increases. This disadvantage associated with myocardial immaturity is especially important during the initial transitional period. As the low-resistance placental circulation is removed, SVR suddenly increases. This acute rise in the SVR and afterload may compromise left cardiac output and systemic blood flow. Indeed, superior vena cava (SVC) flow, used as a surrogate for systemic blood flow (left cardiac output), is low in a large proportion of VLBW infants during the first 6 to 12 postnatal hours. The exaggerated decrease in myocardial contractility in response to increases in left ventricular afterload may play a role in development of low SVC flow. However, this low flow state appears to be transient, because the majority of the patients recover by 24 to 36 hours after delivery. Similarly, findings of Doppler studies of CBF suggest an increase in CBF shortly after delivery. Therefore, although the myocardium of the preterm neonate undergoes structural and functional maturation over many months, it appears that, after a transient dysfunction of varying severity immediately after delivery, it can relatively rapidly adapt to the postnatal changes in systemic hemodynamics.
In addition to the developmentally regulated susceptibility to dysfunction, a decrease in the oxygen supply associated with perinatal depression is a major cause of poor myocardial function and low cardiac output in preterm and term neonates immediately following delivery. During fetal life, despite being in a “hypoxic” environment by postnatal norms, neuroendocrine and other compensatory mechanisms and the unique fetal circulation enable the fetus to tolerate the “relative” hypoxemia and even brief episodes of true fetal hypoxemia. Indeed and as mentioned earlier, during fetal hypoxemia the distribution of blood flow is altered to maintain perfusion and oxygen supply to the vital organs, including the heart. However, a significant degree of hypoxemia, especially when associated with metabolic acidosis, can rapidly exhaust the compensatory mechanisms and result in myocardial dysfunction. The critical threshold of fetal arterial SaO 2 below which metabolic acidosis develops varies depending on the cause of fetal hypoxia. In the animal model of maternal hypoxia-induced fetal hypoxia, fetal arterial oxygen saturations less than 30% are associated with metabolic acidosis. In addition, it appears that the fetus is more or less susceptible to hypoxia if the cause of hypoxia is umbilical cord occlusion or decreased uterine blood flow, respectively. Human data obtained by fetal pulse oximetry are consistent with the results of animal studies and indicate that the SaO 2 of 30% is indeed the threshold for the development of fetal metabolic acidosis.
An increase in cardiac enzymes and cardiac troponin T and I are useful in the assessment of the degree of myocardial injury associated with perinatal asphyxia. In addition, increases in cardiac troponin T and I have been shown to be helpful in diagnosing myocardial injury even in the mildly depressed neonate. Although cardiac troponin T and I may be more sensitive than echocardiographic findings in detecting myocardial injury, the cardiovascular significance of the elevation of troponins in the absence of myocardial dysfunction remains unclear.
Modifications of the cardiac contractile protein myosin regulatory light chain 2 (MLC2) has also been implicated in the development of cardiac systolic dysfunction following newborn asphyxia. In a piglet model of perinatal asphyxia, a decrease in MLC2 phosphorylation and an increase in MLC2 degradation via nitration were observed, suggesting that these are potential targets for therapeutic interventions to reduce myocardial damage in perinatal depression. Nevertheless, documentation of clinical relevance of these findings is necessary to determine the future utility of such therapies.
Tricuspid regurgitation is the most common echocardiographic finding in neonates with perinatal depression and myocardial dysfunction. In cases with severe perinatal depression and myocardial injury, myocardial dysfunction frequently leads to decreases in cardiac output and the development of full-blown cardiogenic shock. Finally, if the myocardium is not appropriately supported by inotropes, the ensuing low cardiac output will exacerbate the existing metabolic acidosis (see Chapter 28 ).
Cardiogenic shock due to congenital heart defect, arrhythmia, cardiomyopathy, and PDA is discussed in other chapters in this book.
Vasodilation
The regulation of vascular smooth muscle tone is complex and involves neuronal, endocrine, paracrine, and autocrine factors ( Fig. 1.3 ). Regardless of the regulatory stimuli, intracellular calcium availability plays the central role in regulating vascular smooth muscle tone. In the process of smooth muscle cell contraction, the regulatory protein calmodulin combines with calcium to activate myosin kinase. This enzyme phosphorylates the myosin light chain, facilitating its binding with actin and thus resulting in contraction. As for vasodilation, in addition to the reduction in intracellular calcium availability, myosin phosphatase generates muscle relaxation by dephosphorylation of the myosin light chain.
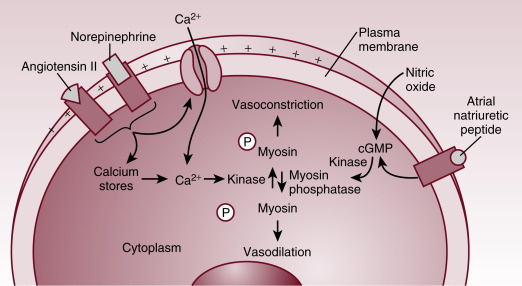
Maintenance of the vascular tone depends on the balance between the opposing forces of vasodilation and vasoconstriction. The vasodilatory and vasoconstricting mediators exert their effects by inducing alteration in cytosolic calcium concentration and/or by direct activation of the enzymes involved in the process. Influx of calcium through cell membrane voltage-gated calcium channels and release of calcium from sarcoplasmic reticulum are the two sources responsible for the rise in cytosolic calcium required for muscle contraction.
The role of various potassium channels has been identified in the regulation of vascular tone. Among these, the adenosine triphosphate (ATP)-dependent potassium channel (K ATP ) emerged as the key channel through which many modulators exert their action on vascular smooth muscle tone. In addition, the K ATP channel has been implicated in pathogenesis of vasodilatory shock. K ATP channels are located on the smooth muscle cell membrane, and opening of these channels leads to K + efflux with resultant hyperpolarization of the cell membrane. Cell membrane hyperpolarization in turn causes the closure of the voltage-gated calcium channels in the cell membrane and thus a reduction in cytosolic calcium and a decreased vascular tone. Under normal conditions the K ATP channels are closed for the most part. However, under pathologic conditions a number of stimuli may activate these channels and thus affect tissue perfusion. For instance, via the associated reduction in ATP and the increase in H + concentration and lactate levels, tissue hypoxia activates K ATP channels resulting in vasodilation and a compensatory increase in tissue perfusion.
As mentioned earlier, a number of vasodilators and vasoconstrictors exert their effects through the K ATP channels. For example, in septic shock, several endocrine and paracrine factors such as atrial natriuretic peptide, adenosine, and NO are released resulting in activation of K ATP channels. Thus K ATP channels are thought to play an important role in pathogenesis of vasodilatory shock. Indeed, animal studies have shown an improvement in BP following administration of K ATP channel blockers. Inhibition of K ATP channels can improve vascular tone in vasodilatory shock; however, because K ATP channels are ubiquitously expressed and involved in a number of cellular and tissue functions, their inhibition can also lead to adverse effects. A small human trial failed to show any benefit of administration of the K ATP channel inhibitor glibenclamide in adults with septic shock. Although the sample size was small and several problems have been identified with the methodology, the findings of this study support the notion that the mechanism of vasodilation in septic shock is more complex than initially believed (see later).
Eicosanoids are derived from cell membrane phospholipids through metabolism of arachidonic acid by the cyclooxygenase or lipoxygenase enzymes and have a wide range of effects on vascular tone. For example, prostacyclin and prostaglandin E 2 (PGE 2 ) are vasodilators, whereas thromboxane A 2 is a vasoconstrictor. Apart from their involvement in the physiologic regulation of vascular tone, these eicosanoids also play a role in pathogenesis of shock. Both human and animal studies have shown a beneficial effect of cyclooxygenase inhibition in septic shock. In addition, rats deficient in essential fatty acid and thus unable to produce significant amounts of eicosanoids are less susceptible to endotoxic shock than their wild-type counterparts. However, the role of eicosanoids in the pathogenesis of shock is also more complex and some studies suggest that, under different conditions, they may actually have a beneficial role. For example, administration of prostaglandin I 2 (PGI 2 ), PGE 1 , and PGE 2 improves the cardiovascular status in animals with hypovolemic shock. Another layer of complexity is revealed by the observation that production of both the vasodilator and vasoconstrictor prostanoids is increased in shock.
NO is another paracrine substance, which plays an important role in the regulation of vascular tone. Normally, NO is produced in vascular endothelial cells by the constitutive enzyme endothelial NO synthase (eNOS). NO then diffuses to the adjacent smooth muscle cells, where it activates guanyl cyclase, resulting in increased cyclic guanosine monophosphate (cGMP) formation. cGMP then induces vasodilation by the activation of cGMP-dependent protein kinase and the different K + channels, as well as by the inhibition of inositol triphosphate formation and calcium entry into the vascular smooth muscle cells.
In septic shock (see Chapter 27 ), endotoxin and cytokines such as tumor necrosis factor alpha result in increased expression of inducible NO synthase (iNOS). Studies in animals and humans have shown that the NO level significantly increases in various forms of shock, especially in septic shock. This excessive and dysregulated production of NO then leads to severe vasodilation, hypotension, and vasopressor resistance (see Chapter 30 ). Because of the role of NO in the pathogenesis of vasodilatory shock, a number of studies have looked at the NO production pathway as a potential target of therapeutic interventions. However, studies using a nonselective NOS inhibitor in patients with septic shock have found significant side effects and increased mortality associated with this treatment modality. The deleterious effects were likely due to inhibition of eNOS, the constitutive NOS that plays an important role in the physiologic regulation of vascular tone. Indeed, subsequent studies in animal models using a selective iNOS inhibitor found an improvement of BP and a reduction in lactic acidosis. Finally, results of studies on the use of nonselective NOS inhibitors in adult humans with septic shock have also not been favorable.
There has been a renewed interest in the cardiovascular effects of vasopressin. Although in postnatal life and under physiologic conditions, this hormone is primarily involved in the regulation of osmolality, and there is accumulating evidence suggesting a role of vasopressin in the pathogenesis of vasodilatory shock. Vasopressin exerts its vascular effects through the two isoforms of V 1 receptors. V 1a receptor is expressed in all vessels, whereas V 1b is present only in the pituitary gland. The renal epithelial effects of vasopressin are mediated through V 2 receptors.
Postnatally and under physiologic conditions, vasopressin contributes little if any to the maintenance of vascular smooth muscle tone. However, under pathologic conditions such as in shock, the decrease in BP vasopressin production increases, attenuating the further decline in BP. However, with progression of the circulatory compromise, vasopressin levels decline as pituitary vasopressin stores become depleted. The decline in vasopressin production leads to further losses of vascular tone and contributes to the development of refractory hypotension. Findings on the effectiveness of vasopressin replacement therapy in reversing refractory hypotension further support the role of vasopressin in the pathogenesis of vasodilatory shock. The vasoconstrictor effects of vasopressin appear to be dose dependent. As mentioned earlier, excessive production of NO and activation of K ATP channels are some of the major mechanisms involved in the pathogenesis of vasodilatory shock. Under these circumstances, vasopressin inhibits NO-induced cGMP production and inactivates the K ATP channels, resulting in improvement in vascular tone. In addition, vasopressin releases calcium from sarcoplasmic reticulum and augments the vasoconstrictive effects of norepinephrine. As for its clinical use, vasopressin has been shown to improve cardiovascular function in neonates and children presenting with vasopressor-resistant vasodilatory shock after cardiac surgery. However, the few published case series on preterm infants with refractory hypotension show variable effects of vasopressin treatment with improvement in BP and urine output only in some patients. A pilot study showed similar efficacy of vasopressin to dopamine in improving BP and signs of shock in preterm infants. Although vasopressin was associated with somewhat less tachycardia, the clinical significance of this finding is unclear. It is clear that more data are needed before vasopressin can be used routinely in the neonatal population. In addition, a meta-analysis and trial sequential analysis concluded that vasopressin does not reduce mortality or intensive care unit stay in refractory shock and may increase the risk of ischemic injury in the pediatric population.
In general, vasodilation with or without decreased myocardial contractility is the dominant underlying cause of hemodynamic disturbances in septic shock. However, there are very limited data on changes in cardiovascular function in neonates with septic shock. A study in preterm infants with late-onset sepsis found that the high cardiac output characteristic for the earlier stages of septic shock diminished and SVR sharply increased before death in nonsurviving patients, whereas there was only a mild increase in the SVR during the course of the cardiovascular disturbance in patients who survived. The authors also described a significant variability in hemodynamic response among the survivors. In children, two distinct presentations of shock are seen: one with vasodilation (warm shock) characterized by high CO and low SVR and the other with vasoconstriction (cold shock) characterized by high SVR and low CO. A study in children with fluid resistant shock found different patterns of hemodynamic derangement in central venous catheter-related (CVCR) versus community-acquired (CA) infections. Low SVR and low cardiac output were the dominant pathophysiologic findings in patients with CVCR and CA septic shock, respectively. These findings suggest that the hemodynamic response may be different depending on the type of bacterial pathogen and/or represent the fact that patients with CA septic shock are usually diagnosed at a later stage and thus myocardial dysfunction might have already set in at the time of the diagnosis. Although vasodilatory shock is thought to be the main presentation of neonates with septic shock, a recent study in preterm infants provided some evidence suggestive of the prevalence of vasoconstrictive type shock. In older children, approximately 50% of patients with septic shock present with either systolic or diastolic dysfunction. However, it is not known how common myocardial dysfunction is in the neonatal population with septic shock. The results of the previous studies underscore the importance of direct assessment of cardiac function by echocardiography and tailoring the treatment strategy according to the hemodynamic finding in each individual patient.
The case study presented next underscores this point and illustrates that the population-based BP values defining hypotension must be viewed as guidelines only and do not necessarily apply to an individual patient. This is explained by the fact that a number of factors including gestational and postnatal age, preexisting insults, partial pressure of carbon dioxide in the arteries and PaO 2 levels, acidosis, and the underlying pathophysiology all impact the critical BP value in the given patient at which perfusion pressure becomes progressively inadequate to first sustain vital organ (brain, heart, adrenal glands) perfusion and blood flow autoregulation, then brain function, and, finally, structural integrity of the organs.
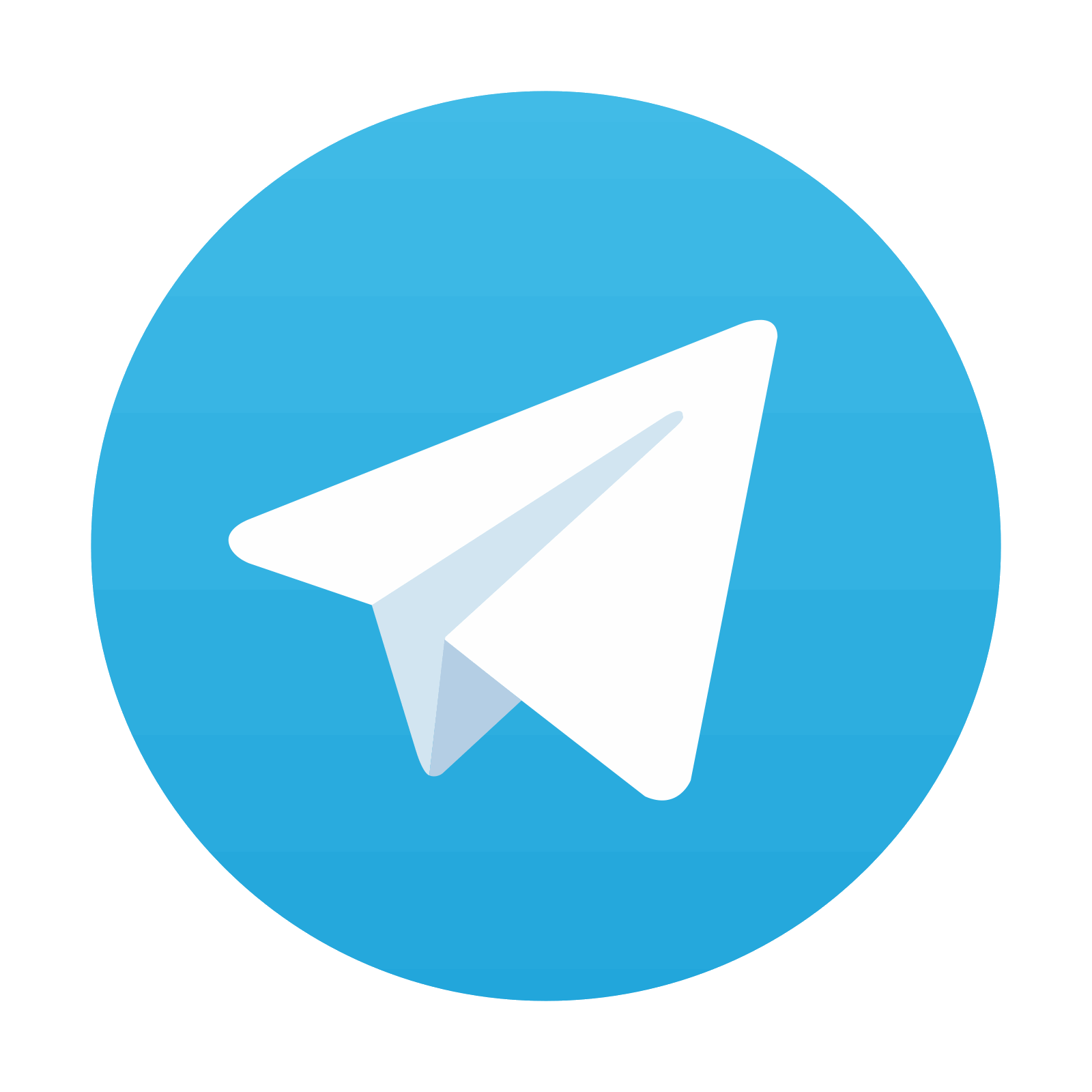
Stay updated, free articles. Join our Telegram channel
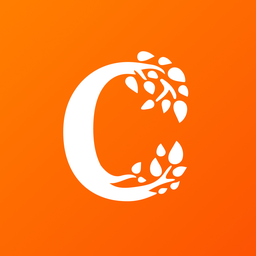
Full access? Get Clinical Tree
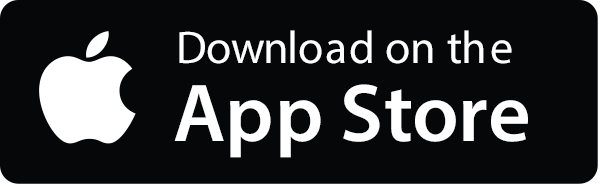
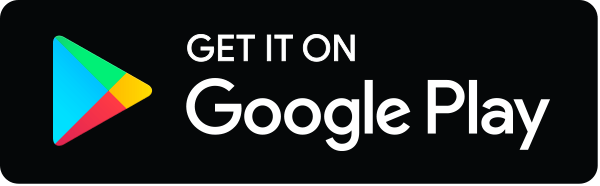