Radiopharmaceuticals for Clinical PET, PET-CT, and SPECT-CT Imaging
Gustav K. von Schulthess
Designing good radiopharmaceuticals for imaging is a more difficult task than one might think. It is therefore not surprising that equipment manufacturers change the technology they offer almost on a yearly basis, while the introduction of a new radiopharmaceutical may take 5 years or more from its first appearance in the literature. In general, a contrast agent or radiopharmaceutical should provide high sensitivity, relatively high specificity, and low toxicity. Agents that are too specific are typically too costly for clinical use, as their development cost can be recovered from employing them with only a small number of patients. They nevertheless may eventually prove their value as we move into the age of personalized medicine, where such expensive tracers may prevent giving even more expensive drugs to a patient.
Although toxicity restrictions essentially do not exist for radiopharmaceuticals, many aspects of their design are still tricky. The number of radioisotopes available is limited, and the PET radiopharmaceuticals in widespread use will predominately be 18F based. Too short a half-life makes a radioisotope impractical for performing chemistry on it, too long a half-life leads to unacceptable radiation doses to the patient, particularly when part of the emission is in beta particles, as is the case in PET. Radiopharmaceuticals need to be relatively easy to synthesize and have good kinetic properties, binding strongly but not to strongly, for example, to the receptor intended for study (see Chapter 13). Furthermore, since only the label and not the pharmaceutical is seen in the nuclear imaging process, the radiopharmaceutical should not be metabolized extensively. It turns out that in regard to many of these issues FDG has excellent properties.
Nuclear imaging, be it conventional nuclear imaging or PET imaging, requires radiation sources in the form of radioisotopes alone or bound into biomolecules. These labeled biomolecules participate in physiological processes and thereby become “metabolic spies.” The “art” of radiopharmacy is thus to identify molecules that are suitable for labeling a given metabolic process, synthesize them reliably, and, in the case of PET, where the short half-lives of the radioisotopes drastically limit the time of their usefulness, synthesize them in the shortest possible time. This chapter outlines some general principles of contrast enhancement and discusses considerations regarding the design of radiopharmaceuticals.
Basic Principles of Contrast Enhancement in Imaging
In general, external contrast enhancement in imaging is used to enhance the contrast between structures and their background. Except in the case of nuclear imaging, this enhancement is superimposed on the contrast already existing between various body structures (i.e., structures are already seen on the images), but the external contrast media make the structures more conspicuous. In nuclear imaging, the situation is completely different, in that the human body contains virtually no radioactivity. Thus, no structures in the body are seen without the administration of a nuclear contrast agent, which in nuclear imaging is called a “radiopharmaceutical.” The potential advantage of this unique situation is that a very high lesion-to-background or structure-to-background ratio can be achieved, typically causing the lesion or structure to be very conspicuous in the image. In nuclear imaging, the radiopharmaceutical provides the entire contrast; in the other medical imaging modalities, external contrast merely adds some contrast in most instances.
Radiopharmaceuticals also differ entirely from the contrast agents used in other imaging modalities in the dose range at which they have to be injected to produce adequate contrast in the images (see Table 1.1).
Whereas in ultrasound, CT, and MR, the concentration of contrast agents needed to affect the images is in the 1 mmol to the 10 μmol range, nuclear imaging operates at radiopharmaceutical concentrations 10,000 10,000,000 times lower. (For the sake of completeness, optical imaging, whose properties are similar to those of nuclear imaging, is also listed in Table 1.1, but due to difficulties of transmission of light through the body, optical imaging so far can only be used in small animal imaging experiments.) This difference in the effective concentration of contrast agents and radiopharmaceuticals is critical. In nuclear imaging, no physiological or pharmacological side effects are observed after the injection of a radiopharmaceutical, except in the rare case of extremely toxic receptor imaging agents. Thus, allergic and other reactions to radiopharmaceuticals are not observed. This is in stark contrast to the contrast agents used in other imaging modalities, where adverse reactions are known to occur after their injection. Consequently, most of the molecular mechanisms that can be targeted with radiopharmaceuticals are inaccessible to imaging with the contrast agents used in the other modalities. Thallium, which was in routine use in nuclear cardiology, is rat poison, but in myocardial perfusion scanning it was given at a concentration 50,000 times lower than the LD50. Compounds such as Tc-MIBI and Tc-Myoview could in principle also be labeled with gadolinium, but the resulting MR contrast agent would be too toxic for use. The same is true of fluorodeoxyglucose, as the stable 19F isotope is amenable to fluorine-MRI. Although it is possible to amplify the MR signal by using, for example, small ferrite particles linked to biomolecules, such complexes have very unfavorable accumulation biokinetics. Thus, “molecular imaging” and “nuclear imaging” are currently synonymous in clinical practice. This is important to understand given the current “hype” about molecular imaging engulfing MRI.
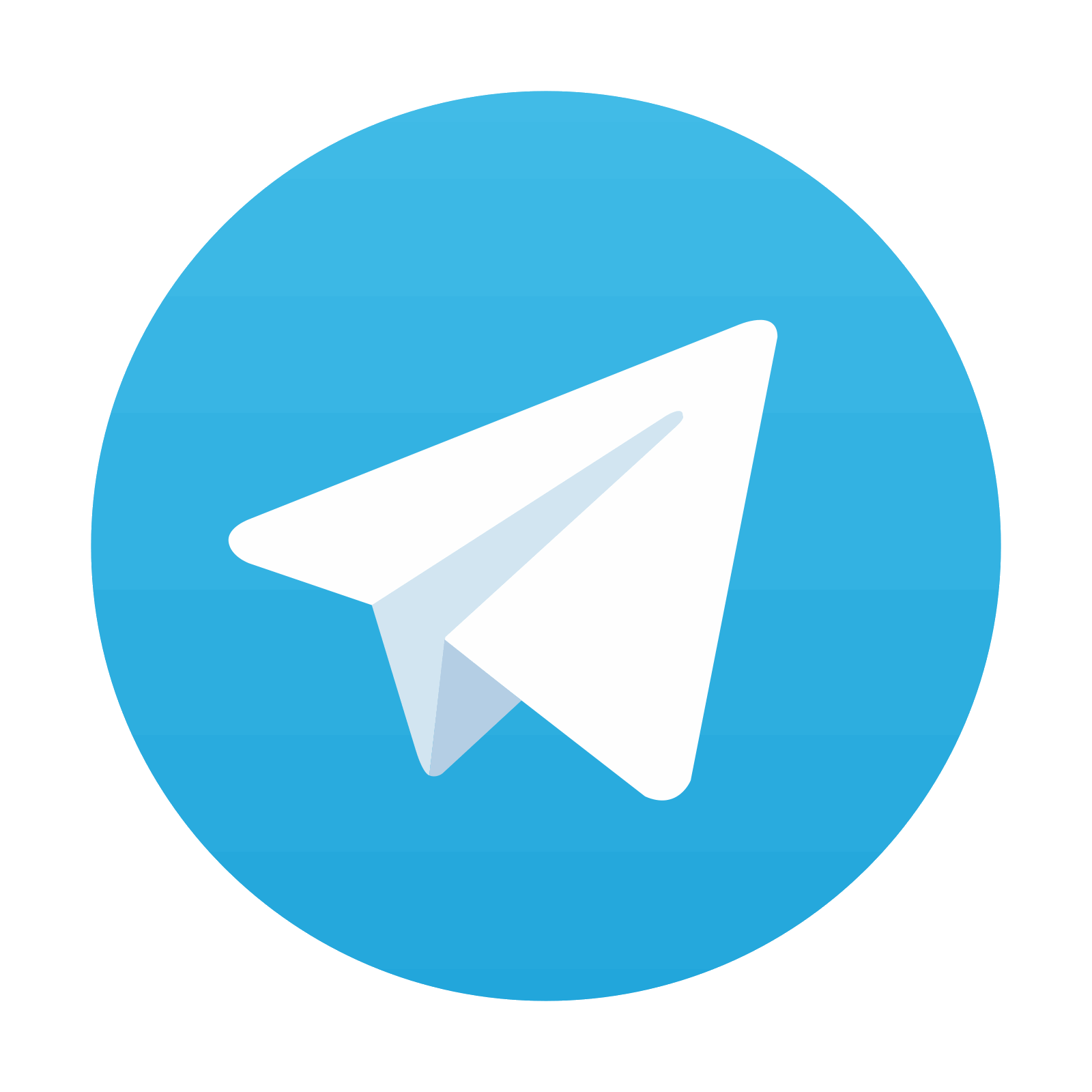
Stay updated, free articles. Join our Telegram channel
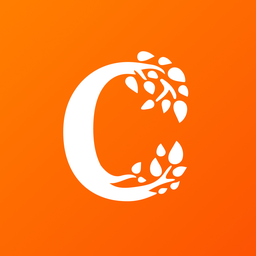
Full access? Get Clinical Tree
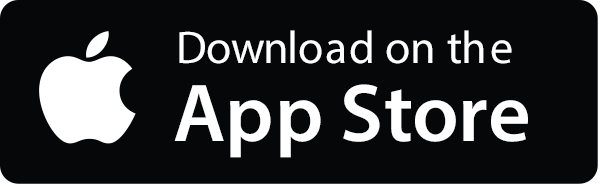
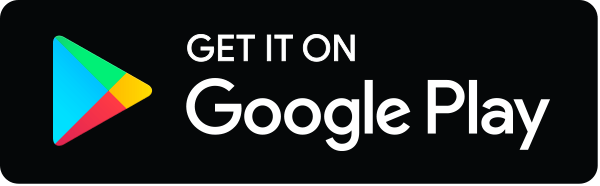