Fig. 1
Chemical structures of the corticosteroid receptor agonist and antagonist ligands discussed
This chapter will explore the structural determinants of function within the corticosteroid receptors, primarily focusing on the MR but drawing analogy with the GR as appropriate, noting also that the GR is extensively discussed in Chap. 3 of this volume. Critical to understanding structure-function relationships in the MR are the interactions made both internally and with other factors (Yang and Fuller 2012) , many of which will influence the conformation of the MR or depend on a specific conformation.
2 Mineralocorticoid Receptor Structure
The human MR (NR3C2) is the longest of the nuclear receptors containing 984 amino acids, by contrast the human GR (NR3C1) contains 777 amino acids. As with other members of the NR family, the MR has three major functional domains: an N-terminal domain (NTD), a central DNA-binding domain (DBD) and a C-terminal ligand-binding domain (LBD) (Arriza et al. 1987). The MR-NTD (602 amino acids) is the longest of the steroid hormone receptors and it shares little sequence homology with these other receptors (Lavery and McEwan 2005) . The MR-NTD contains three regions to which an activation function (AF) has been ascribed: AF1a (amino acids 1-169), MD (middle domain, amino acids 247–385) and AF1b (amino acids 451–602) (Fischer et al. 2010; Fuse et al. 2000; Govindan and Warriar 1998; Pascual-Le Tallec et al. 2003) . A central inhibitory region (amino acids 163–437) has also been identified (Pascual-Le Tallec et al. 2003) although it may reflect the ability of the MD to recruit corepressors (Fischer et al. 2010). Vlassi et al. (2013) report that the inhibitory domain (amino acids 168–445 in the human MR) contains 15 tandem repeats of ~ 10 amino acids, an ensemble that is highly conserved in evolution and is not observed in the GR. The authors predict that these repeats will form a β-solenoid surface which may play a role in dimerisation or intermolecular hydrophobic interactions of MR. Aside from regulating transactivation, the NTD also interacts with the LBD as discussed below (Rogerson and Fuller 2003) . The NTD is considered to be intrinsically disordered in the absence of binding partners allowing structural flexibility for diverse protein interactions (McEwan et al. 2007) . AF-1b has recently been shown to adopt a stable secondary structure and can interact with protein targets in the absence of induced folding (Fischer et al. 2010) , highlighting the complexity of this region .
The DBD of 66 amino acids is highly conserved across the nuclear receptor superfamily . It contains two zinc ions tetrahedrally coordinating four cysteine residues and residues important for DNA recognition and binding, as well as for receptor homo- and hetero-dimerisation (Pippal and Fuller 2008) . It is linked to the LBD by a hinge region of 61 amino acids that may play a role in receptor dimerisation (Savory et al. 2001) .
The LBD has a canonical structure that is conserved across the NR superfamily (Tsuji 2013) . The MR LBD of 251 amino acids is organised in eleven αhelices (labelled by convention 1–12; helix 2 is unstructured in the SHRs) and four β-strands forming three anti-parallel layers (Bledsoe et al. 2005; Fagart et al. 2005; Huyet et al. 2007; Li et al. 2005) . It contains a ligand-dependent activation function 2 (AF-2) made up of helices 3, 4, 5 and 12. The LBD interacts with chaperone proteins in the absence of ligand and undergoes conformational change upon ligand binding to form AF-2, a hydrophobic cleft on the surface of the LBD which binds coregulators .
3 Structural Determinants of Ligand-Specificity
Within the steroid receptor sub-family of the nuclear receptor superfamily (GR, MR, AR and PR), the amino acid sequence of the ligand-binding domains share 50–60 % identity. This sequence conservation has a functional correlate in that the MR antagonist spironolactone is also an antagonist at the AR and agonist at the PR, progesterone is an antagonist at the MR and GR, while RU486 is a PR and GR antagonist, yet all is not promiscuity. To understand the structural basis of how the MR is able to bind aldosterone and cortisol , yet the GR binds only cortisol, Rogerson et al. (1999) took a chimeric approach. Such approaches had previously been used to explore the structural basis of differences in ligand-binding affinity for the same receptor across species (Benhamou et al. 1992; Keightley et al. 1998) . Sixteen MR:GR chimeric LBD were created with the three break points being at regions of high identity, the study having been initiated prior to publication of the crystal structures of the GR (Bledsoe et al. 2005) and MR (Bledsoe et al. 2002; Fagart et al. 2005; Li et al. 2005) . Despite this, the structural integrity was preserved in that all chimeras were able to bind cortisol albeit with a spectrum of affinities. The full- length receptor containing the LBD chimeras was expressed in CV-1 cells with an MMTV-reporter in a conventional transactivation assay. The N-terminus and DBD were derived from the GR, a strategy based largely on the fact that the GR N-terminus is more active in a transactivation assay than the N-terminus of the MR (the reverse is true of the respective LBD) (Rupprecht et al. 1993; Lim-Tio et al. 1997) . Of the 16 chimeras, those that contained MR sequences in the second segment (amino acids 804–870) were both transcriptionally active and bound aldosterone (Rogerson et al. 1999) . Curiously this was not true for cortisol where transactivation only occurred when the second and fourth regions were derived from the same receptor, i.e. both MR or both GR; this clearly argues for a difference in the LBD interactions between aldosterone and cortisol. The region of MR 804–874 also conferred binding specificity on spironolactone (Rogerson et al. 2003) and eplenerone (Rogerson et al. 2004) neither of which bind with significant affinity to the GR. Rogerson et al. (2007) subsequently used the same approach with smaller regions of MR 804–874 to identify a 25 amino acid region (MR 820–844) containing four critical residues that together confer the ability to bind or not bind aldosterone on the MR and GR respectively (Fig. 2).
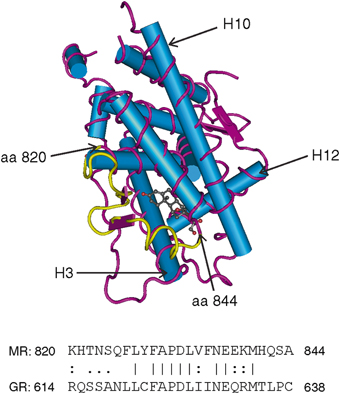
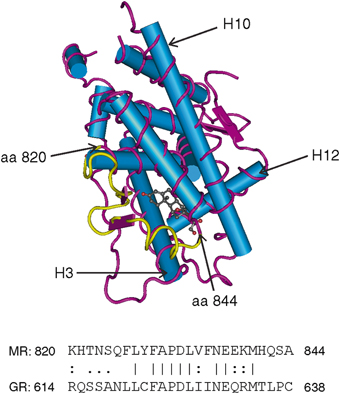
Fig. 2
MR LBD crystal structure with the region MR820-844 highlighted. α-helices are represented by the rods and β-sheets as ribbons (based on Bledsoe et al. 2005—Protein Data Bank 2AA2). Helices 3, 10 and 12 are labelled as such. Below is the amino sequence for the 820–844 region of the MR and the equivalent region of the GR which is primarily a loop between helicies 5 and 6
Other studies using AR:PR chimeras (Vivat et al. 1997) , GR:PR chimeras (Robin-Jagerschmidt et al. 2000) and MR:GR chimeras (Martinez et al. 2005) identified regions responsible for ligand specificity that correspond to the MR 820–844 region. Amino acids 820–844 cover parts of helix 5, helix 6 and the loop between. Only one amino acid, the phenyalanine at position 829 in the MR has been described as contributing to the ligand-binding pocket of the MR LBD (Bledsoe et al. 2005) ; however this amino acid is also found at the equivalent position in the GR. Amino acids 820–844 are otherwise predicted to lie on the surface of the LBD, particularly the H5-H6 loop. This finding is reminiscent of our findings for the guinea-pig GR, which relative to the human GR, is resistant to cortisol due to a five amino acid difference in the region between H1 and H3, which is another unstructured loop predicted to be on the surface of the GR LBD (Bledsoe et al. 2002). These observations are consistent with the notion that it is an external interaction of these regions that mediates ligand sensitivity and selectivity.
4 Chaperone Proteins
In the absence of ligand, MR (and GR) are complexed with cytoplasmic chaperone proteins (Rafestin-Oblin et al. 1989) , including the 90 kDa (hsp90), which is crucial for high-affinity ligand-binding by the MR (Binart et al. 1995; Huyet et al. 2012) . The MR is also known to interact with the 70 kDa (hsp70), small acidic protein p23 and tetratricopeptide repeat (TPR, 34 amino acids repeated in tandems)-domain proteins such as FK506-binding proteins (FKBPs), cylophilins (CyPs) or serine/threonine protein phosphatase 5 (PP5) (Bruner et al. 1997; Pratt and Toft 1997; Rafestin-Oblin et al. 1989) . This complex maintains the MR in an appropriate conformation for high-affinity ligand- binding and prevents its nonspecific activation (Faresse et al. 2010) . The other co-chaperones play a dynamic role in determining ligand affinity (Huyet et al. 2012). Binding of hsp90 to the MR is mediated, at least in part, by the C-terminal of the hinge region (Huyet et al. 2012). The absence or antagonism of hsp90 leads to the polyubiquitylation and proteasomal degradation of MR via the ubiquitin-protein ligase, COOH-terminus of hsp70-interacting protein (CHIP) (Faresse et al. 2010).
Dissociation of hsp90 and other chaperone proteins from the MR is thought to take place in the cytoplasm upon aldosterone binding prior to the nuclear translocation of MR. However evidence has shown that hsp90 is actually required for the efficient nuclear transport of the MR by linking it to the FKBP52-dynein motor protein complex (Galigniana et al. 2010a, b) . This large heterocomplex most likely passes intact through the nuclear pore since the MR can be recovered bound to hsp90 immediately after nuclear translocation while MR transformation appears to occur within the nucleus 10–15 min after steroid binding. Grossman et al. (2012) have recently demonstrated that MR homodimerisation occurs in the nucleus when agonist is bound rather than in the cytoplasm. FKBP51, which does not bind dynein and negatively regulates MR action, dissociates from the MR upon aldosterone binding so as to permit the recruitment of FKBP52 (Galigniana et al. 2010b; Gallo et al. 2007) . This differential regulation of dynein interaction by FKBP51 and FKBP52 is also seen with the GR (Wochnik et al. 2005) . The cochaperone SGTA (small glutamine-rich tetratricopeptide repeat-containing protein alpha) represses receptor activity for AR, PR and GR through an interaction with FKBP52, yet is without effect on the MR (Paul et al. 2014) . In the case of the GR, it has been well established that the relative concentrations of FKBP51 and FKBP52 will determine the affinity of the unliganded receptor for steroid with FKBP52 promoting a higher binding affinity. It is these interactions for instance that appear critical to the reduced binding affinity seen for the guinea pig GR (Cluning et al. 2013) . This argues that a helix 1-helix 3 loop interaction with FKBP51/52 plays a role in defining the conformation and hence the ligand-binding affinity of the unliganded GR (Cluning et al. 2013).
These observations reinforce a critical limitation in our understanding of the structure of steroid receptors given the lack of a crystal structure for the unliganded receptor. Whilst there is good evidence from other nuclear receptors for a critical shift in helix 12 with a “tighter” conformation of the helices when ligand is bound, the current crystal structure provides only a snapshot of the “final resting place”. The critical interaction between steroid and unliganded receptor is not captured, which is an inherent limitation to any attempt at rationale drug design.
5 Structural Determinants of Agonism Versus Antagonism
The classical MR antagonist spironolactone is seen as a “passive antagonist” where competition for binding occurs but the conformation of the LBD is largely unaltered, and the internal conformation changes needed for transactivation fail to occur (Bledsoe et al. 2005) . Spironolactone is in fact a weak agonist/predominant antagonist; the agonist response can be enhanced by cyclic AMP analogues, in a cell and promoter dependent context and by MR coactivators (Nordeen et al. 1995; Massaad et al. 1997; Rogerson et al. 2014) . Eplerenone, the other MR antagonist currently in clinical practice, is a derivative of spironolactone and as such exhibits a similar mechanism of action. Although eplerenone has a lower affinity for the MR than spironolactone, it is much more selective, being almost devoid of activity at the other steroid receptors (Fagart et al. 2010) .
Insights into the structural basis of the antagonism have been drawn from the observations that a single serine to leucine substitution at position 810, which was identified in a kindred with hypertension (Geller et al. 2000; Zhang et al. 2005) , made spironolactone and progesterone agonists as it does the normally inactive metabolite of cortisol , cortisone (Rafestin-Oblin et al. 2003) . Zhang and Geller (2008) argue that the helix 3-helix 5 interaction is critical both in the MR and across steroid receptors. These helices contain residues which form hydrogen bonds with the steroid A and D rings (the latter also interacts with residues in helix 12) to bind the steroid with high affinity. This positioning of the steroid in the binding pocket with the critical points of contact being at both ends of the steroid, holding the steroid rather like a “chicken in a rotisserie” as first noted with the estrogen receptor α (ERα) (Brzozowski et al. 1997) . Zhang and Geller (2008) have argued that the Ser 810 Leu mutation results in an enhanced ligand-independent interaction of helix 3 and helix 5 promoting transactivation by otherwise antagonist ligands, a postulate that is at least partially supported by subsequent solution of the crystal structure of the MR LBD containing these and other mutations (Fagart et al. 2005; Bledsoe et al. 2005; Li et al. 2005) . Curiously spironolactone can also be rendered agonist by changes in other amino acids. For both the trout and zebra-fish MR, spironolactone (and progesterone) are agonist, yet they do not contain a substitution at Ser810 (Sturm et al. 2005; Pippal et al. 2011) . Whilst this helix 3-helix 5 interaction is clearly of considerable importance, these studies and indeed other structural analyses are confounded by several limitations: (1) there is a tendency to focus on a specific region, e.g. the helix 5–6 loops (Rogerson et al. 1999; 2007) , the helix 3–5 interactions (Geller et al. 2000; Zhang et al. 2005; Zhang and Geller 2008) etc., yet all are potentially relevant and perhaps can’t be viewed in isolation; (2) binding is somehow taken as being synonymous with transactivation yet these can clearly be dissociated (Rogerson et al. 1999), indeed antagonism may be seen as a dissociation of binding and transactivation while absence of binding renders any other considerations null and void; (3) although the data derived from the published MR LBD crystal structures are of enormous value (Bledsoe et al. 2005;, Li et al. 2005; Fagart et al. 2005) , they all contain various LBD mutations to facilitate formation of stable crystals (Zhang and Geller 2008), which results in an agonist conformation irrespective of the ligand; (4) specificity and sensitivity in the context of binding are arguably the same thing, i.e. specificity purely reflects differences in affinity; and (5) in the context of agonism versus antagonism the studies tend to overlook (Zhang and Geller 2008) the importance of the interactions formed with helix 12 as highlighted by Auzou et al. (2000) in which correct positioning of helix 12 is absolutely essential for the AF-2 function and its consequent interaction with coactivator molecules.
6 Interdomain Interactions
Despite a view which arose from the early studies following the cloning of the steroid receptors (Green and Chambon 1987) that the primary domains are largely modular and can be “mixed and matched”, it is now clear that interdomain interactions may be critical to function. A ligand-dependent interaction between the N-terminal domain and the C-terminus/LBD has been extensively characterised for the AR (Langley et al. 1995; He et al. 1999; Zhou et al. 1995) . This interaction has been shown to be fundamental to AR function, indeed mutations causing androgen insensitivity syndrome in humans have been identified that selectively abrogate the N/C-interactions (Thompson et al. 2001; Quigley et al. 2004) . An N/C-interaction has also been described for the PR (Tetel et al. 1999) and ERα (Mètivier et al. 2002) . Some years ago we identified an equivalent ligand-dependent interaction for the MR (Rogerson and Fuller 2003) . Curiously this interaction is not seen for the GR. This aldosterone-induced interaction is antagonised by spironolactone and eplerenone and rather surprisingly by cortisol and deoxycorticosterone, clear evidence that these MR agonists induce a different conformation in MR LBD to that induced by aldosterone . The interaction is conserved across evolution in that it is also observed with the zebra fish MR (Pippal et al. 2011) . Studies using fluorescence response energy transfer (FRET) have shown for the AR that the interaction can be intramolecular when the AR is a monomer and intermolecular when it is a dimer (Klokk et al. 2007; Schaufele et al. 2005) . In the AR, FxxLF-like motifs in the N-terminus bind to the AF-2 region of the AR LBD competing for binding of the classical LxxLL-motif found in steroid receptor co-activators. The structural determinants appear to be different for the MR in that although the interaction is a direct protein-protein interaction between the N- and C-terminal domains (Pippal et al. 2009) , the MR N-terminal domain lacks FxxLF or LxxLL motifs and indeed deletion studies suggest more than one region is involved (Pippal et al. 2009) . Four sumoylation sites identified in the human MR (3 of which are conserved in the zebra fish MR) do not compromise the N/C-interaction when inactivated by mutating the lysines to arginine. The unstructured nature of the N-terminal domain, where composition rather than sequence may be more important, makes the dissection of the interactions particularly challenging. On the C-terminal side, inactivation of AF-2 function attenuates but does not eliminate the N/C-interaction (Rogerson and Fuller 2003) , this is in contrast to the interaction of the MR LBD with LxxLL motif containing coactivators where the interaction is completely eliminated (Rogerson et al. 2014).
7 DNA Binding
The primary mode of action of MR is as a transcription factor with its DBD binding to a hormone response element (HRE) in the promoter of target genes to mediate transcription (Lombes et al. 1993) . Specific HREs have been characterized for ER, PR, GR and AR, but a selective MR response element has yet to be described. That the MR interacts with diverse GR response elements is not surprising in that it shares 94 % identity with the GR DBD (Arriza et al. 1987; Funder 1993; Lombes et al. 1993) . These HREs typically consist of two receptor binding half-sites with the consensus seuences 5’-TGTTCT-3’ arranged as an inverted palindrome separated by three nucleotides (Luisi et al. 1991) although two novel HREs upstream of the endothelin-1 gene have been described for the MR and GR that are half-sites separated by eight nucleotides (Stow et al. 2009) . The MR has also been shown to interact directly with the promoter of the epidermal growth factor receptor (EGFR) via a region that does not contain the canonical GRE (Grossmann et al. 2007) .
The sequence of the specific HRE also plays a role in determining the response. The GRE has been shown to regulate GR conformation to determine selective cofactor interactions (Meijsing et al. 2009) . This also has been reported for the ER (Heery et al. 1997; Loven et al. 2001) and AR (Brodie and McEwan 2005) with their respective HRE. The response element bound by the AR has been shown to induce conformational changes in the NTD (Brodie and McEwan 2005; Geserick et al. 2003) . The HRE is therefore an important interacting partner with unique regulatory functions. Whether the same applies to the MR remains to be determined.
In addition to binding as homodimers to HRE, the MR is also capable of forming heterodimers with other steroid receptors, in particular the GR, to offer additional transcriptional control. Trapp et al. (1994) demonstrated a direct MR-GR interaction with a dissociation rate of the heterodimer that was slower than either of the homodimers resulting in a synergistic effect on transcription activation. By contrast Liu et al. (1995) reported that MR/GR heterodimers inhibited transcription. Inhibition of the neuronal serotonin receptor in response to corticosterone was greater for MR/GR heterodimers than for MR or GR homodimers alone (Ou et al. 2001) . The GR-LBD has been shown to be required for heterodimerisation with the MR (Savory et al. 2001) . Coordinated signaling by GR and MR may be important in tissues such as the hippocampus where there is an abundance of both receptors, and a recent study highlighted the potential role of MR and GR heterodimerisation in the kidney (Ackermann et al. 2010). Co-localisation of the MR and GR was observed in all cell types of the aldosterone-sensitive distal nephron and they underwent distinct patterns of subcellular localisation in response to corticosteroids (Ackermann et al. 2010) . MR-GR heterodimers have been detected by FRET in hippocampal cells where the level of heterodimerisation is regulated by fluctuations in cortisol concentrations in response to circadian rhythm and stress (Nishi 2010).
Repression of gene expression is well characterised for other steroid hormone receptors, either by competition with other transcription factors for overlapping DNA binding sites or by direct protein-protein interaction in a DNA-independent manner (Fig. 3) (Cato et al. 1992) . The GR for example mediates transcriptional repression through a direct interaction with activator protein 1 (AP-1) and nuclear factor kappa B (NF-κB) (Jonat et al. 1990; Ray and Prefontaine 1994) . The C-terminal zinc finger of the MR-DBD, which is identical to the GR, is reported to also inhibit NFκB by a direct interaction with its RelA subunit (Liden et al. 1997) . Reciprocal inhibition of MR-mediated transcription by NFκB has been observed (Kolla and Litwack 2000) , however the physiological relevance of these observations remains to be determined. In other systems the MR appears to enhance NFκB and AP-1 activity (Fiebeler et al. 2001) . Conversely, the MR is unable to repress AP-1 induced transactivation (Cato et al. 1992; Pearce and Yamamoto 1993) . These differences in MR and GR transactivation at the same response element lead Pearce (1994) to speculate that “transcription of genes linked to putative composite MREs is either activated or repressed depending on the composition of nonreceptor factors bound at the elements”.
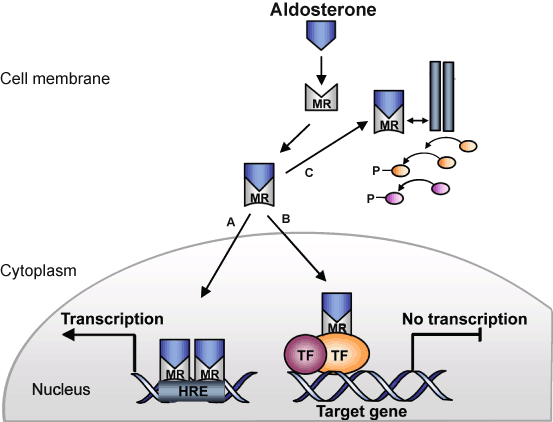
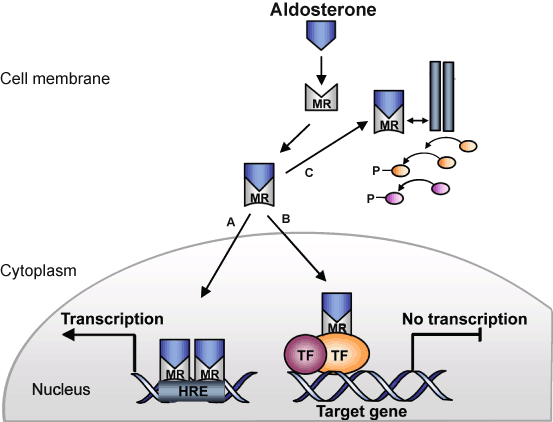
Fig. 3
Schematic of MR signalling. Aldosterone binding to the cytoplasmic MR leads to a conformational change and nuclear transfer where the MR regulates gene expression through binding to a specific response element (HRE) and interactions with coregulating molecules (a). An alternative putative mechanism of MR-mediated gene regulation (b) involves interaction with other transcription factors (TF). Rapid signalling involves an interaction of the MR with other signalling pathways at the cell surface to trigger second messenger mediated signalling pathways (c)
8 Co-Activators and Co-Repressors
Once bound to the regulatory region of a target gene, the liganded-receptor complex is interacting with both the chromatin and the transcriptional apparatus through coregulatory molecules which are often part of a larger transcriptional complex (McInerney et al. 1998) . These complexes perform many of the functions needed for gene expression, including chromatin remodelling, histone modification, initiation of transcriptional elongation of RNA transcripts and termination of transcription (Auboeuf et al. 2007; O’Malley 2007) . Over 400 coregulators have been described for the nuclear receptor superfamily (Bulynko and O’Malley 2011) although to date less than 20 have been described for the MR (Yang and Young 2009; Yang et al. 2012) . The well characterised coactivators steroid coactivator-1 (SRC-1), SRC-2, SRC-3 and the peroxisome proliferator-activated receptor gamma coactivator 1-alpha (PGC-1α) have all been shown to interact with the AF-2 region of the MR LBD via classical LxxLL motifs as they do for the other receptors. In no case do they show ligand-discriminant transactivation. Indeed two studies which explore interactions between known coactivator-derived LxxLL motifs and the MR LBD using either a mammalian-2 hybrid assay (Hultman et al. 2005) or an alpha-screen (Li et al. 2005) found a relatively small number of interactions, all of which interacted in the presence of both cortisol and aldosterone. In contrast, using a yeast-2-hybrid screen with the MR LBD, we identified a novel LxxLL motif-dependent MR coactivator, tesmin, that shows ligand-discriminant transactivation for aldosterone versus cortisol in that under identical conditions, the cortisol-mediated transactivation by the MR is not enhanced by the presence of tesmin yet it is for aldosterone (Rogerson et al. 2014) . We have also identified MR interacting proteins using phage-display with and without LxxLL motifs which are ligand-discriminant (Yang et al. 2011; Yang et al. 2014) . Although these studies again highlight the difference in the conformation of the MR LBD induced by cortisol and aldosterone, the structural determinants of these differences remain to be determined.
Other groups have sought MR-specific coactivators by screening with the N-terminal region. A yeast two-hybrid screen of a human kidney cDNA library using the MR-NTD as bait identified the elongation factor ELL (eleven-nineteen lysine-rich leukemia) as an MR-specific interacting partner (Pascual-Le Tallec et al. 2005) . ELL interacts exclusively with the AF-1b region of the MR and is selective in potentiating MR-mediated transactivation while repressing GR transactivation. ELL is co-expressed with the MR in the cortical collecting duct cells of the human kidney and is rapidly upregulated by aldosterone (Pascual-Le Tallec et al. 2005). This may be relevant in tissues with coexpression of both receptors (Bookout et al. 2006) . Ubiquitin-like protein SUMO-1 (small ubiquitin-related modifier-1) conjugating enzyme (Ubc9), is involved in the sumoylation process whereby Ubc9 interacts with the MR-NTD and potentiates aldosterone-dependent MR transactivation independent of its sumoylation activity (Yokota et al. 2007) . SRC-1 is recruited simultaneously and can synergistically potentiate MR-mediated transcription with Ubc9, suggesting that Ubc9 mediates its effect by binding SRC-1 and recruiting other coactivators. This is supported by the co-localisation of MR, Ubc9 and SRC-1 in mouse kidney collecting duct cell nuclei (Yokota et al. 2007) .
Protein inhibitor of activated signal transducer and activator of transcription 1 (PIAS1), another sumoylation enzyme , has also been found to interact with the MR-NTD (Pascual-Le Tallec et al. 2003) . PIAS1 significantly inhibits aldosterone-dependent MR-mediated transactivation. The repressive effect of PIAS1 is partly mediated by sumoylation of the MR. PIAS1 contains three LxxLL motifs, all of which have been shown to enhance transactivation mediated by the AR and GR (Tan et al. 2000) . PIAS3, like PIAS1, also interacts with the MR-NTD and represses MR-induced transactivation in a neural cell line (Tirard et al. 2004) . Differential modulation of MR- and GR-mediated transcription by PIAS1 and PIAS3 offers another mechanism by which the two receptors can achieve receptor-specific effects.
The earliest identified corepressors, nuclear receptor corepressor (NCoR) and silencing mediator of retinoid and thyroid hormone receptor (SMRT) , interact with the MR-LBD via their CoRNR boxes and have been shown to attenuate aldosterone-dependent MR-mediated transactivation by inducing histone deacetylase activity (Wang et al. 2004) . These corepressors were identified through their role in transcriptional repression of nuclear receptors or when bound to synthetic antagonists in the absence of ligand (Chen and Evans 1995; Horlein et al. 1995) . However, recent data has shown that NCoR is recruited by the MR upon aldosterone binding but not upon binding of spironolactone, a steroidal MR antagonist, or BR-4628, a newly synthesised non-steroidal MR antagonist (Fagart et al. 2010) .
NF-YC is a subunit of the heterotrimeric transcription factor NF-Y which recognises a CCAAT box motif found in the RNA polymerase II initiation site in many eukaryotic promoters and activates transcription (Nakshatri et al. 1996) . It interacts with the MR-NTD and represses MR transactivation in a dose- and agonist-concentration dependent manner (Murai-Takeda, et al. 2010) . The mechanism of repression may involve disruption of the N/C-interaction. NF-YC has no effect on GR, AR or PR-mediated transactivation.
9 Post-Translational Modification
Post-translational modifications such as receptor phosphorylation, sumoylation and ubiquitination (Fig. 4) have been described as significant modulators of NR signaling by altering their protein-protein interactions, DNA binding and degradation, although information relating to the MR is sparse (Viengchareun et al. 2007) .
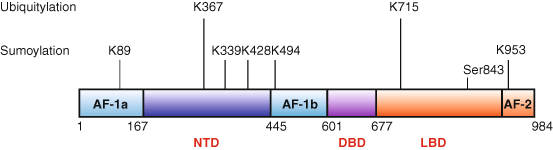
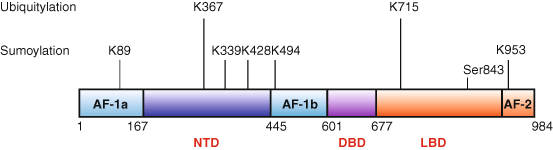
Fig. 4
Post-translational modifications of the MR. The human MR sequence is shown with domains (N-terminal domain—NTD; DNA-binding domain-: DBD; ligand binding domain—LBD) indicated, as are the positions of the described activating functions (AF). The lysine (K) residues subject sumoylation and ubiquitylation are indicated as is serine 843 which is inactivated by phosphorylation (Shibata et al. 2013). A more extensive list of phosphorylation sites of uncertain biological significance can be found in Shibata et al. (2013)
Phosphorylation is ubiquitous among all eukaryotic cellular proteins and critically regulates protein structure, localisation and stability (Lalevee et al. 2010) . The MR contains tyrosine, serine and threonine residues in its NTD and LBD which represent potential phosphorylation sites (Hirschberg et al. 2004; Viengchareun et al. 2007) . In this context, the MR 820–844 region has been brought into focus by a recent study which reports that phosphorylation of serine 843 in the human MR inactivates the MR (Shibata et al. 2013) They argue for a physiological role for serine 843 phosphorylation where in the intercalated cells of the distal nephron but not the principal cells, hyperkalaemia increases phosphorylation through an unspecified kinase. Conversely angiotensin II promotes sodium and hydrogen ion flux by increasing dephosphorylation of serine 843. The authors propose that this allows a degree of dissociation of the two feedback loops mediated by aldosterone through the MR: (i) volume homeostasis through the renin-angiotensin system which increases aldosterone synthesis and hence sodium retention; and (ii) potassium homeostasis where hyperkalaemia promotes aldosterone synthesis with consequent renal potassium secretion. Whilst sodium retention and potassium secretion are coupled in the principal cells, this is not the case in the intercalated cells.
Mutations in the serine/threonine-rich sequences of the MR nuclear localisation signal suggest a role for phosphorylation in receptor subcellular shuttling (Walther et al. 2005) . An interaction between the MR and protein kinase D1 (PKD1) modulates the subcellular trafficking of the MR and stabilises its nuclear localisation (McEneaney et al. 2010) . Cyclin-dependent kinase 5 (CDK5) has also been shown to phosphorylate three serine and threonine residues within the NTD of the MR in an aldosterone-dependent fashion to suppress MR transcriptional activity (Kino et al. 2010) .
Sumoylation involves the covalent attachment of small ubiquitin-related modifiers (SUMO) to the lysine residues of substrate proteins. It is catalysed by a cascade of enzymes including the E1-activating enzyme, E2-conjugating enzyme Ubc9 and E3-ligase (Seeler and Dejean 2003) . This process occurs at the consensus motif ψKxD/E (ψ is a hydrophobic residue, K is the lysine targeted by SUMO-1, x is any amino acid and D/E is an acidic residue). These residues were originally identified as the “synergy control motif” in the GR-NTD which acted as a negative regulatory element of GR transactivation (Iniguez-Lluhi and Pearce 2000) . Via the same motif, sumoylation of the AR, PR and GR has repressive effects on their transactivation (Abdel-Hafiz et al. 2002; Poukka et al. 2000b; Tian et al. 2002) . The MR contains the most number of sumoylation sites, with four in the NTD and one in the LBD (Poukka et al. 2000a) which interacts with components of the sumoylation machinery , including Ubc9 (SUMO-1 conjugating enzyme) and SUMO-1 (Tirard et al. 2007) . Ubc9 has previously been identified as a coactivator of the MR, independent of its sumoylation ability (Yokota et al. 2007) . In contrast, SUMO-1 binding mediates repression of transcriptional synergy at the MR while sumoylation deficient MR displayed reduced nuclear mobility and therefore longer periods of promoter occupancy and transcriptional activation (Tirard et al. 2007). The exact biological significance of sumoylation is unclear but the highly conserved nature of these motifs and their presence in the negative regulatory regions of many transcription factors suggest they play an important functional role in controlling receptor activity (Viengchareun et al. 2007) .
Ubiquitylation and proteasomal degradation have been reported for the MR (Tirard et al. 2007; Yokota et al. 2004) but again the functional significance is unknown. The GR undergoes proteasomal degradation and clearance from the nucleus at the trough of each corticosterone pulse within hippocampal cells but the MR remains intact in the nucleus (Conway-Campbell et al. 2007) . This may be a further method of differential regulation of the GR and MR in tissues where they are coexpressed.
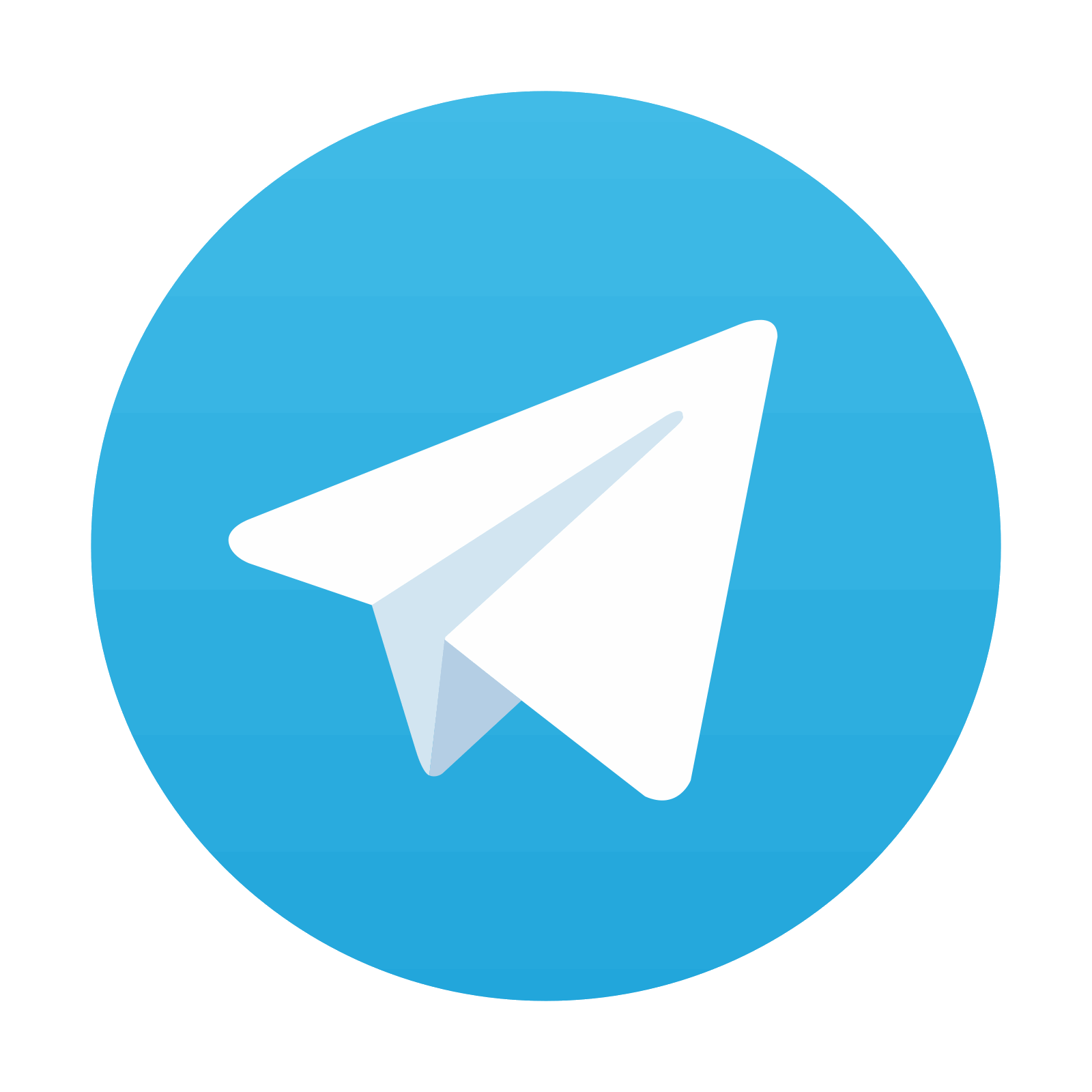
Stay updated, free articles. Join our Telegram channel
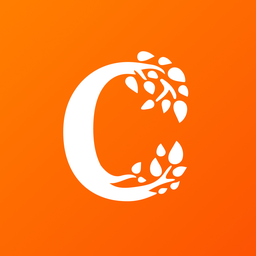
Full access? Get Clinical Tree
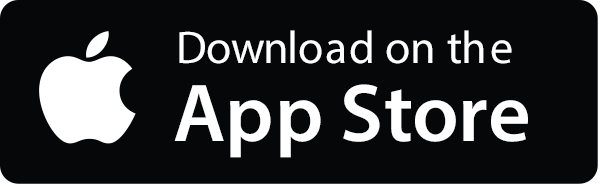
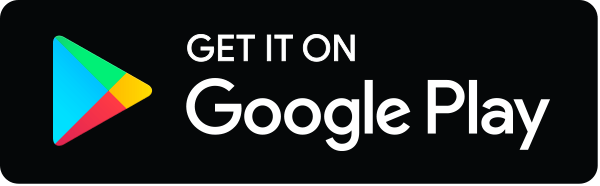