Fig. 1
The domain organization of NHR’s The diagram reads N-terminus on the left, C-terminus on the right. The N-terminal domain (NTD) is intrinsically disorder and often binds cofactor proteins. The DNA-binding domain (DBD) binds response elements. The ligand-binding domain (LBD) binds small molecules, such as steroids, and some cofactor proteins. The NTD length is highly variable and its sequence is poorly conserved. The DBD and LBD together are on the order of 300 amino acids and are well conserved
The LBD contains several dynamic regions that are predominantly near the ligand-binding pocket. Some of the earliest research on the dynamic nature of the LBD is from the O’Malley lab in the 1990’s. Working with progesterone and estrogen receptors, they found that the LBD undergoes a conformational change upon binding hormone (Allan et al. 1992a, 1992b; Beekman et al. 1993) . Subsequent X-ray crystallography and fluorescence anisotropy experiments demonstrated that when the LBD binds ligand, helix-12 undergoes a conformational change and becomes less dynamic (Bourguet et al. 1995; Renaud et al. 1995; Wagner et al. 1995; Kallenberger et al. 2003) . This conformational change varies depending on the ligand bound; hence different ligands can promote specific conformations that bind corepressors or coactivators (Brzozowski et al. 1997; Nahoum et al. 2007) . Researchers have also shown that cofactor fragments that bind the LBD can stabilize the LBD interaction with certain ligands (Gee et al. 1999; Kallenberger et al. 2003) , demonstrating an allosteric communication between cofactor and ligand binding. The cofactor thus acts as an allosteric effector/ligand.
The DBD also has regions that are dynamic. Estrogen and glucocorticoid receptor DBDs are partially disordered in solution (Berglund et al. 1997; Schwabe et al. 1990) , and become more ordered when bound to DNA (Schwabe et al. 1995; Luisi et al. 1991) . Spolar and Record first described this transition on thermodynamic grounds (Spolar and Record 1994) , and their analysis hinted at the possibility of different DNA sequences having different effects on the DBD. Indeed, the sequence of DNA does modulate this conformational change (Bain et al. 2012; Meijsing et al. 2009; Loven et al. 2001) . Specifically, the DNA sequence can change both the binding affinity to the DBD and the propensity for dimerization of the DBD (Hudson et al. 2013; Wood et al. 2001; Glass et al. 1988) . It has been speculated that response elements cause allosteric effects beyond the DBD—possibly changing the conformation of the NTD (Lefstin et al. 1994; Starr et al. 1996) , a point that will be revisited in the next section .
The steroid hormone receptor (SHR) subclass of NHRs contains large NTDs of varying size and sequence, which are perhaps the best example of dynamics and disorder in the NHR family of proteins (Krasowski et al. 2008) . Each SHR type contains a large amount of ID in the N-terminus, as determined by a myriad of biophysical methods (Fischer et al. 2010; Dahlman-Wright et al. 1995; Kumar and Thompson 2010; Bain et al. 2000, 2001; Lavery and McEwan 2008; Nocula-Ługowska et al. 2009) . It is well-established that many disordered proteins undergo coupled folding and binding to perform their biological role (Uversky et al. 2005) . In 1999, two manuscripts presented the first evidence that the NTD of an SHR can fold into a tertiary structure, either because of the DBD binding a response element or because of high concentrations of an osmolyte (Baskakov et al. 1999; Kumar et al. 1999) . When so folded, the NTD showed enhanced binding of known partner proteins (Kumar et al. 2001). Since then, many groups have used various osmolytes, such as trimethyl amine-N-oxide (TMAO; see Bolen and Baskakov 2001) to fold the NTDs of NHRs and have demonstrated that they fold cooperatively, a hallmark of a naturally evolved protein (Watters et al. 2007) .
Glucocorticoid , androgen, and mineralocorticoid receptors’ NTDs all fold cooperatively in the presence of molar concentrations of TMAO (Reid et al. 2002; Li et al. 2012; Fischer et al. 2010; Baskakov et al. 1999) . This is evidence of a major folded conformation or an ensemble of folded conformations. The folded state is the presumed biologically active state of the NTD, and this is supported by studies showing the NTD folds upon binding transcriptional cofactors. The NTDs of SHRs bind numerous cofactor proteins (Lavery and McEwan 2005) . Studies on estrogen, glucocorticoid, and androgen receptors have shown that the conformational ensembles of the NTDs become more ordered upon binding cofactor proteins (Reid et al. 2002; Copik et al. 2006; Warnmark et al. 2001; Khan et al. 2012; Kumar et al. 2004) . This suggests a coupled folding and binding mechanism that regulates SHR activity.
In sum, all NHR domains—even the relatively structured LBDs and DBDs—are dynamic and exist as conformational ensembles of states. Upon binding a ligand, domains are stabilized in one or another globular state of a more limited ensemble. These ligand-specific effects have functional consequences on the co-regulators bound, and on transcriptional function. By varying the transcriptional function of a receptor, ligands can alter cellular and clinical outcomes. “Ligand” thus refers to all binding partners—protein, DNA, steroidal or other small molecules—as they interact with their respective domains.
2 Inter- and Intra-Domain Coupling in NHRs
Binding of a ligand in one NHR domain not only affects the conformational ensemble of that domain, but also the conformational ensembles of the other domains. This allosteric coupling between and within the major domains is crucial for the function of the receptors.
2.1 DNA Response Elements as Allosteric Effectors
Early glucocorticoid research raised a fundamental question (Ivarie and O’Farrell 1978) —how does a NHR activate a multitude of genes to different degrees? One possibility is that each response element could modify the activity of a bound NHR. This idea was supported by research on glucocorticoid, estrogen, and thyroid hormone receptors (Lefstin et al. 1994; Allan et al. 1992b; Glass et al. 1988; Starr et al. 1996; Sakai et al. 1988; Martinez and Wahli 1989) . In particular, work from Yamamoto and colleagues demonstrated that glucocorticoid receptor activity was dependent on the response element sequence (Sakai et al. 1988) and also speculated that a conformational change was occurring upon DNA binding.
Ikeda and colleagues provided the first evidence of different response elements inducing different conformational changes in NHRs (Ikeda et al. 1996) . They found that transcriptionally active response elements induced a change in thyroid hormone/retinoid X receptor dimers, such that the complex was resistant to protease digestion, relative to protein dimers on inactive response elements (Ikeda et al. 1996) . Later studies found similar effects with estrogen and androgen response elements and their cognate receptors (Wood et al. 1998, 2001; Geserick et al. 2003; Loven et al. 2001) .
The limited proteolysis experiments described above demonstrate DNA response element dependent protection patterns. However, which portions of the molecule were undergoing conformational changes was still an open question. In 1999, Kumar and colleagues published the first manuscript demonstrating that when an NHR, glucocorticoid receptor, binds a site specific DNA its NTD undergoes a conformational change (Kumar et al. 1999) . Kumar used a combination of circular dichroism and tryptophan fluorescence to demonstrate that folding was occurring in the NTD. Subsequent studies have revealed similar phenomena in progesterone receptor (Bain et al. 2000, 2001) , estrogen receptor alpha (Greenfield et al. 2001) , and androgen receptor (Brodie and McEwan 2005) . The inferred significance of these observations is that binding of DNA coupled to folding of the NTD would recruit transcriptional cofactors, as proposed by Thompson and coworkers (Copik et al. 2006; Thompson and Kumar 2003) .
2.2 LBD Ligands as Allosteric Effectors
Different ligands binding to the LBD can elicit specific transcriptional responses. Early results on this matter are conflicting. Several studies suggested that binding of hormone increased the binding affinity for DNA (Bagchi et al. 1988; Yamamoto and Alberts 1972; Becker et al. 1986) while others did not (King and Greene 1984; Welshons et al. 1984) . Some of these ambiguities are likely due to different DNA response elements used by different labs. As shown by Meyer and coworkers, different response elements can modulate the effect of a given hormone. They demonstrated that RU486, a known antagonist, of the progesterone receptor A isoform could activate the B isoform. Furthermore, this activation only occurred on one of the two promoter sequences that they tested (Meyer et al. 1990) . This is an example of selective response modification by an NHR, in which a ligand modifies receptor activity in a manner dependent on the DNA bound (Gerber et al. 2009) . By this and probably other mechanisms, selective sets of genes are activated or repressed by specific steroids acting through their cognate receptors. Such ligands activate distinct, but usually overlapping sets of genes.
Selective response modifiers exert their effects through a number of mechanisms. Besides affecting DNA binding, ligands also change subsequent events. Different ligands alter recruitment of NTD binding partners to estrogen and glucocorticoid receptors (Ronacher et al. 2009; Garside et al. 2004; Shang and Brown 2002) . Furthermore, ligands can also alter dimerization of NHRs, as seen in the formation of PPARα-RXRα heterodimers on DNA (Forman et al. 1997) . Regardless of the variety of mechanisms, what is clear from these examples is that ligand binding at the LBD causes allosteric effects on the DBD and NTD.
2.3 Splicing and Translational Isoforms Modulate Allosteric Communication
As do many proteins, NHRs can exist in multiple isoforms (Lu and Cidlowski 2005; Cao et al. 2013; Tontonoz et al. 1994; Schrader and O’Malley 1972; Talbot et al. 1993) , and several studies have demonstrated that NHR isoforms differ in their transcriptional activities (Mouillet et al. 2001; Lu and Cidlowski 2005; Meyer et al. 1990) . However, it is rarely made clear how isoforms have different transcriptional activities or specificities. One possibility is that each isoform contains a unique system of allosteric coupling and disorder. This notion is supported by recent work showing that tissue-specific coding exons are enriched in intrinsic disorder and binding motifs (Buljan et al. 2012) . Interestingly, NTDs of NHRs are often intrinsically disordered and full of co-regulator binding sites (Krasowski et al. 2008) . This raises the possibility that tissues tune the activity of a NHR by expressing varying amounts of the NTD isoforms.
Three steroid receptors are known to possess alternative NTD isoforms (progesterone, glucocorticoid, and ecdysone receptors; see: Kastner et al. 1990 ; Lu and Cidlowski 2005; Talbot et al. 1993) . Of the three ecdysone receptor isoforms, two have very similar NTD lengths and yet vastly different effects on transcriptional activity (Mouillet et al. 2001; Dela Cruz et al. 2000) . Similar effects have been reported for progesterone and glucocorticoid receptors (Meyer et al. 1990; Lu and Cidlowski 2005) , and in the case of glucocorticoid receptor this change in NTD length has an effect on the folding free energy of this disordered domain (Li et al. 2012). Interestingly, the isoform with the lowest folding energy is transcriptionally the most active. This is most readily explained by coupled folding of NTD with binding of coregulators because a more stable isoform will bind to coregulators more strongly.
LBD isoforms also occur. For example, the glucocorticoid receptor has three exon splice-variant isoforms that alter the LBD length (Russcher et al. 2007) . Of these, only GRα is able to bind hormone. The other two (β and γ) have truncated LBDs and markedly different effects on transcriptional regulation (de Lange et al. 2001; Russcher et al. 2005) . Mechanistically, the shorter LBDs ablate the ability of hormone to induce a change in the receptor. However, how these changes affect LBD allosteric coupling to the other receptor domains has not been studied in detail.
In sum, NHRs use inter- and intra-domain coupling to govern their responses to a variety of allosteric effector ligands and thus determine the specificity of their function. Binding of its ligand at any given domain (NTD, DBD, LBD) can affect the stability and binding affinity of their ligands at other domains. These allosteric changes have been understood from a phenomenological view for many years. Only recently has a model been developed that explains these phenomena in testable, quantitative terms. Below we will discuss this general model of allostery (the Ensemble Allosteric Model) that can be used to glean mechanistic insight into NHR allostery.
3 The Ensemble Allosteric Model: Application in the Case of NHRs
How allostery works is a century-old question (Barcroft and Hill 1910; Adair 1925) that was initially addressed with hemoglobin. Monod, Wyman, and Changeux proposed arguably the most influential allosteric model using macroscopic, thermodynamic concepts to take into account a conformational change within the subunits of hemoglobin (Monod et al. 1965) . Other influential models have been proposed that explain most of the available data on hemoglobin (Koshland et al. 1966; Henry et al. 2002) . However, all these models have limitations as they are phenomenological and do not address “how” allostery is mediated between distal sites (Motlagh et al. 2012) . Crystallographic or other structural data has been used to elucidate bond paths linking the binding site of the allosteric effector with a distant responsive site (Perutz et al. 1987; Perutz et al. 1998; M.F. 1970; Suel et al. 2003; Lockless and Ranganathan 1999) . Even though it has been suggested that these allosteric pathways may be dominant (Daily and Gray 2009) , they do not explain the following: all of the available data on hemoglobin (Smith and Ackers 1985) , proteins in which no bond path can be found between the allosteric effector binding site and the distant response region, or the perplexing observation of protein dynamic- and disorder- mediated allostery (Petit et al. 2009; Ferreon et al. 2013; Reichheld et al. 2009) .
We recently proposed a model that alleviates some of these issues by articulating allostery in terms of the intrinsic energetics of a protein—the Ensemble Allosteric Model (EAM) (Hilser and Thompson 2007; Motlagh and Hilser 2012; Hilser et al. 2012; Motlagh et al. 2012) . Because it applies to all proteins, the EAM can be used to describe allostery in NHRs. In particular, it can explain perplexing phenomena, such as the ability of a single ligand to be an agonist or an antagonist to NHR function in different cells (Wolf and Jordan 1992; Amsterdam et al. 2002) .
The EAM is grounded in two well-established observations from protein allostery: The ability of allosteric proteins to exist in multiple conformations in solution, and their segregation of binding sites into different domains. Such domains can communicate with one another, the essence of allostery. From these simple and well-established facts, it is possible to develop the model and ask quantitative questions about allostery.
Consider an allosteric protein consisting of three interacting domains (Fig. 2). The simplest conformational ensemble of each domain is a two state equilibrium between at least one high affinity (H) and low affinity (L) state for its ligand. The L state of a domain can be either intrinsically disordered or an ensemble of somewhat ordered conformers that bind its ligand with lower affinity than does the H conformation. The H and L states for each domain have a free energy difference (i.e. ΔGi), which determines how often the molecule is in the H or L conformation in the absence of influences from other domains. Because the domains communicate to one another, there must be an interaction energy between them. When one domain goes to its L conformation it either stabilizes (i.e. Δg1, 2 < 0) or destabilizes (i.e. Δg1, 2 > 0) the H state of the other domain(s) to which it is coupled (Fig. 2 and Table 1). From this simple articulation, enumeration of all combinations of H and L domain states in addition to their relative free energies is straightforward. Table 1 lists every possible combination of domains 1, 2, and 3 being in either the H or L conformation. The free energy of each state is simply the sum of the conformational energy and the interaction energy. Taking the statistical weight of each state from Table 1 and summing it, yields the partition function (Q) which gives access to every quantity of interest, including experimental observables:
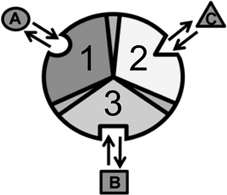
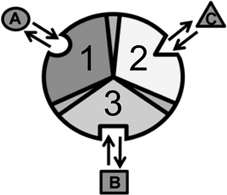
Fig. 2
Cartoon of an allosterically coupled protein with three subunits. The three subunits (1, 2, 3) bind to one ligand each (A, B, C). In the context of NHR’s, the three subunits are the NTD, DBD, and LBD binding to cofactor, DNA, and ligand, respectively. Taken from (Motlagh and Hilser 2012) with permission
Table 1
Breakdown of allosteric states and energies
State | ∑ΔGi | ∑ Δg ,i,j | Si | Probability |
---|---|---|---|---|
HHH | 0 | 0 | 1 | SHHH/Q |
LHH | ΔG1 | Δg12 + Δg13 | K1ϕ12ϕ13 | SLHH/Q |
HLH | ΔG2 | Δg12 + Δg23 | K2ϕ12ϕ23 | SHLH/Q |
HHL | ΔG3 | Δg13 + Δg23 | K3ϕ13ϕ23 | SHHL/Q |
HLL | ΔG2 + ΔG3 | Δg12 + Δg13 + Δg23 | K2K3ϕ12ϕ13ϕ23 | SHLL/Q |
LHL | ΔG1 + ΔG3 | Δg12 + Δg13 + Δg23 | K1K3ϕ12ϕ13ϕ23 | SLHL/Q |
LLH | ΔG1 + ΔG2 | Δg12 + Δg13 + Δg23 | K1K2ϕ12ϕ13ϕ23 | SLLH/Q |
LLL | ΔG1 + ΔG2 + ΔG3 | Δg12 + Δg13 + Δg23 | K1K2K3ϕ12ϕ13ϕ23 | SLLL/Q |

With this, it is possible to reproduce basic allosteric phenomena and ask basic questions. For instance, what happens when ligand A is introduced? The H conformation of each domain will preferentially bind its proper ligand at its introduction into the system (Wyman and Gill 1990) , and introduction of ligand A will stabilize each microstate in Table 1 that has domain 1 in the H conformation by a free energy of:
![$$ \Delta {g_{Lig~A}}= -RTln({1 + {K_{a,A}}[A]}) = -RTln({{Z_{Lig,A}}}) $$](/wp-content/uploads/2016/08/A318767_1_En_5_Chapter_Equb.gif)
In turn, this will change the partition function:

where ZLig,A acts as a weighting term that takes into account the increase in the probability of the H conformation of domain 1 in the presence of A (i.e. ZLig,A = 1 + Ka*[A]). Note that at A = 0 this equation reduces to the original partition function.
Ligand binding to domain 1 changes the statistical weight of some states, but in turn this will change the probability of all states. Of particular interest is the change in probability of the H state of an “active site” (Domain 3; e.g. the binding site for a cofactor protein in the NTD). The probability of domain 3 being in the high affinity, active state without A present is simply the statistical weight of states where domain 3 is in the H state divided by the partition function:
![$$ {P_{3,H}}({[A] = 0}) = \frac{{{S_{HHH}}+ {S_{HLH}}+ {S_{LHH}}+ {S_{LLH}}}}{Q} $$](/wp-content/uploads/2016/08/A318767_1_En_5_Chapter_Equd.gif)
With A present all states with domain 1 in the H state will be redistributed:
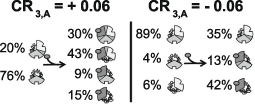
Fig. 3
Examples of CR for single ligand binding. A positive CR3,A indicates that binding of ligand A to domain 1 stabilizes states where domain 3 is in its high affinity conformation. Domains 1, 2, and 3 are displayed as the top left, bottom, and top right of the circle, respectively. The parameters of the positive CR3, A are: ΔG1 = − 1.7, ΔG2 = 2.0, ΔG3 = − 0.9, Δg12 = − 2.3, Δg23 = 0.1, Δg13 = 1.5, and ΔgLig, A = − 5.0 in kcal/mol. The parameters of the negative CR3, A are: ΔG1 = − 2.1, ΔG2 = 1.0, ΔG3 = 1.2, Δg12 = − 1.7, Δg23 = 0.6, Δg13 = − 2.7, and ΔgLig, A = − 5.0 in kcal/mol. From (Motlagh and Hilser 2012) with permission
In this three-domain protein example, one can consider the effect of how an additional ligand, one that binds domain 2 affects the allosteric response of domain 3. When ligand B binds domain 2 it can change the magnitude of the response of domain 3 to ligand A bound in domain 1. The coupling response of other domains to ligand A:Domain 1 binding can change from positive to negative and visa-versa. In a similar manner as before, we can consider the CR of domain 3 when ligand A is added while ligand B is already present:
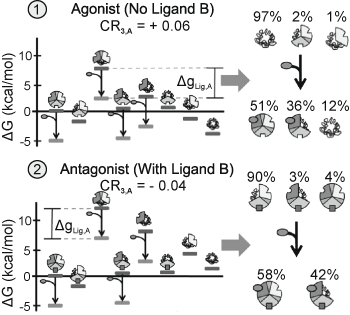
Fig. 4
Positive-Negative response switching. In case ← ligand A acts as a positive regulator of domain 3. In case ↑ the presence of ligand B causes ligand A to act as a negative regulator of domain 3. The parameters used: ΔG1 = − 6.75, ΔG2, B = 0 (case 1) = − 4.4, ΔG2, B > 0 (case 2) = 0.6, ΔG3 = − 2.7, Δg12 = 6.8, Δg23 = 4.8, Δg13 = − 1.9, and ΔgLig,A = − 5.0 kcal/mol. Domains 1, 2, and 3 are displayed as the top left, bottom, and top right of the circle, respectively. From (Motlagh and Hilser 2012) with permission
4 The Ensemble Allosteric Model may Reconcile Puzzling Observations in NHRs
The above model is general and applicable to all allosteric systems, including SHRs. Each of the three domains of an SHR has binding sites for multiple binding partners, similar to the articulation of the EAM. The C-terminal LBD binds small steroidal and synthetic ligands; it also binds a variety of co-regulatory proteins . The central DBD binds DNA sequences and other proteins; DNA acts as a ligand and can have sequence specific effects on transcription and DBD stability. The NTD also binds co-regulators. Application of the EAM to the SHRs at once makes it apparent that all these non-SHR binding partners must be considered ligands, as the preceding discussion explains. Further, the EAM obviously also applies to the two domain group of NHRs (Hilser and Thompson 2007) .
The model has the potential to explain some perplexing observations in NHR research that have important implications for practical applications and drug development. Tamoxifen is known to inhibit breast cancer and yet promote uterine cancer (Wolf and Jordan 1992) , glucocorticoids exert cell-specific anti-inflammatory effects (Amsterdam et al. 2002) , and a number of other NHR’s have effects in a tissue or isoform specific manner (Truman et al. 1994; Walters et al. 2010; Han et al. 2006) . Furthermore, many steroidal and non-steroidal ligands for the LBD act as selective response modifiers (SRMs), meaning that they alter the transcription of selected, though overlapping, sets of genes, in a cell-specific way.
These phenomena may be understood in terms of thermodynamic ensembles. In different cellular contexts, the equilibria of the ensemble could be tuned by perturbations including but not limited to: (1) type and accessibility of DNA response elements; (2) abundance and type of natural/synthetic ligands to LBD; (3) the type and abundance of cofactors to NTD and LBD; (4) different distribution of NHR splicing and translational isoforms; (5) different post-translational modifications to the NHR; (6) effects of intracellular pH, small ion and organic osmolyte levels. All of these will affect the energetic landscape of the ensemble.
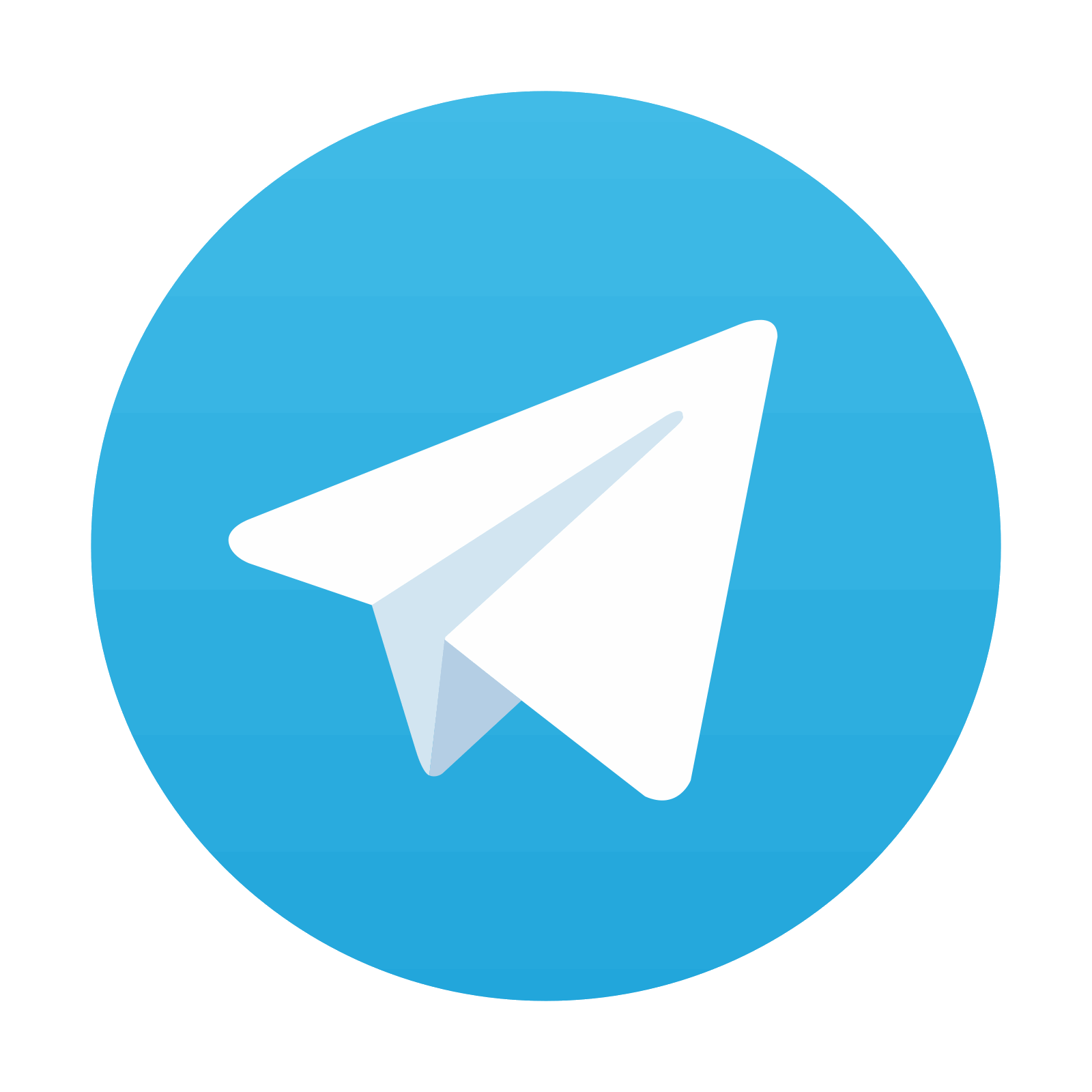
Stay updated, free articles. Join our Telegram channel
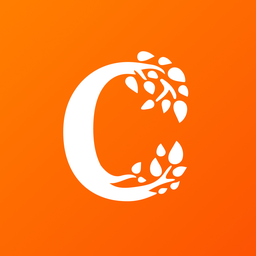
Full access? Get Clinical Tree
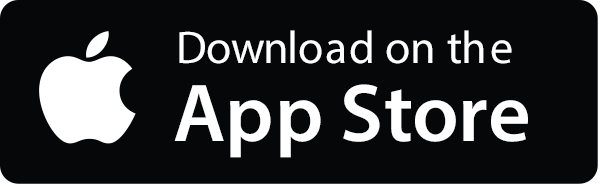
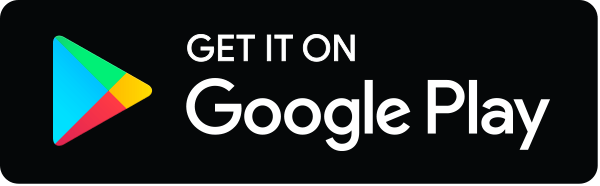
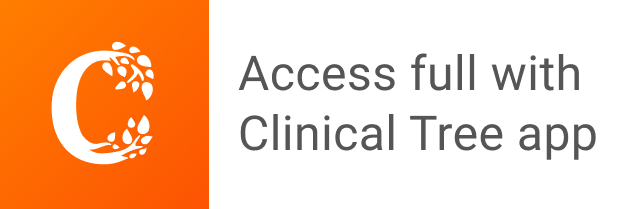