Fig. 1.
(a) Anterior views of LV FE models constructed from subject-specific in vivo MRI data at diastasis. (b) Microstructural images of hearts from 12-months and 24-months WKY and SHR (Adapted from [2, 11]). In the confocal images, brighter intensity reveals the collagen microstructure, while dark gaps indicate the cleavage planes.
Subject-specific microstructural imaging data were not available for the animals used in this study, but LeGrice et al. [2] have previously performed extensive confocal imaging studies to characterise the progression of tissue remodelling in SHRs compared to age-matched WKY rats. They reported that the SHRs exhibited substantial reorganisation of the laminar microstructure (i.e. fusion of sheetlets of myocytes with cleavage space filled with perimysial collagen, and increased endomysial collagen surrounding the myocytes), and that these effects progressed with age (Fig. 1(b)). On the other hand, Pope (2011) reported insignificant differences in the transmural gradients of myocyte fibre orientations both within and between WKY and SHR groups [9]. We, therefore, assumed the fibre angle to vary from -60° on the epicardial surface to +70° on the endocardial surface, and the sheet angle to be +30° throughout the LV models, based on previous histological studies [10, 11].
Following the in vivo MRI experiments, rats were sacrificed and the excised hearts were prepared for ex vivo passive pressure-volume inflation experiments. Each isolated heart was perfused on a Langendorff rig and a balloon was inserted into the LV through the mitral valve. A series of inflation and deflation cycles of the balloon were carried out using a syringe driven at a controlled rate via a pump. The balloon was inflated to reach a specified series of maximum pressures (i.e. 10, 20, and 30 mmHg) in order to pre-condition the balloon. Once this was achieved, an average pressure-volume (PV) curve with maximum LVP up to 30 mmHg was extracted from recordings over several loading cycles. From the averaged curves, we defined the compliance as a function of LV pressure by computing the inverse of the derivative of the PV curve.
2.2 Modelling Passive LV Inflation
To simulate the ex vivo passive pressure-volume inflation experiments, we used the subject-specific 3D LV FE models described in Sect. 2.1. LV mechanics was simulated by solving equations of finite deformation elasticity. The LV base was kinematically constrained, while LV pressure of up to 15 mmHg was incrementally applied to the endocardial surfaces of the models to match the experimental protocols.
2.3 A Constitutive Model of Microstructural Remodelling
To describe the passive mechanical response of the LV, we developed an orthotropic constitutive model (Eq. (1)) of a similar form to that published by Holzapfel and Ogden [12]. In our formulation, we omitted the isotropic term and added an exponential term to independently describe the mechanical response in the sheet-normal (n) direction, and to allow each of the material axes (fibre (f), sheet (s) and sheet-normal (n)) to be separately parameterised. The parameters of this new model (a f , a s , a n , a fs , b f , b n , b s , and b fs ) were initially fitted to ex vivo experimental data describing simple shear of myocardial tissue blocks [13].
To account for the microstructural differences observed between the WKY and SHR myocardium (Fig. 1(b)), we introduced two microstructural parameters (t endo and t peri ) to represent the degree of endomysial and perimysial collagen thickening due to disease progression. Changes in endomysial collagen were postulated to affect the tissue response in the fibre and sheet directions, whereas perimysial collagen was thought to modulate the response in the sheet-normal direction.


(1)
2.4 Myocardial Constitutive Parameters
To investigate the relationships between geometric, microstructural and functional changes in the failing hearts, we estimated the passive constitutive parameters by matching the simulated compliance-pressure curves to the ex vivo experimental data for the 14-months animals. At this age, analyses of high-resolution microstructural images of WKY and SHR myocardium [2] indicated that the endomysial and perimysial collagen were both thicker in the SHR by approximately 5-fold and 10-fold, respectively. Therefore, we set the values of t endo and t peri for the 14-months SHR to be 5 and 10, respectively (note that for the 14-months WKY case, t endo and t peri were both set to 1 as a reference). From the model predicted pressure-volume curves, compliance-pressure relations were derived, and nonlinear optimisation was used to estimate a single set of constitutive parameters (a f , a s , a n , a fs , b f , b n , b s , and b fs ). A nonlinear least-squares algorithm (lsqnonlin in MATLAB1) was used to simultaneously minimise the differences between predicted and experimental compliance data for all subjects.
High resolution microstructural images were not available for the rat hearts at 24-months of age. Therefore, t endo and t peri could not be directly quantified for these older animals. Instead, we tested whether modification of just the microstructural parameters ( t endo and t peri ), while keeping the other constitutive parameters at values fitted to data from the 14-months animals, could reproduce the observed LV chamber compliance curves for the 24-months animals. The goal was to determine whether the modelling framework could yield consistently accurate fits to the functional data from each of the four animals based on the use of subject-specific LV geometries and microstructural parameters (t endo and t peri ), but a single common set of the remaining constitutive parameters in Eq. 1 as described above.
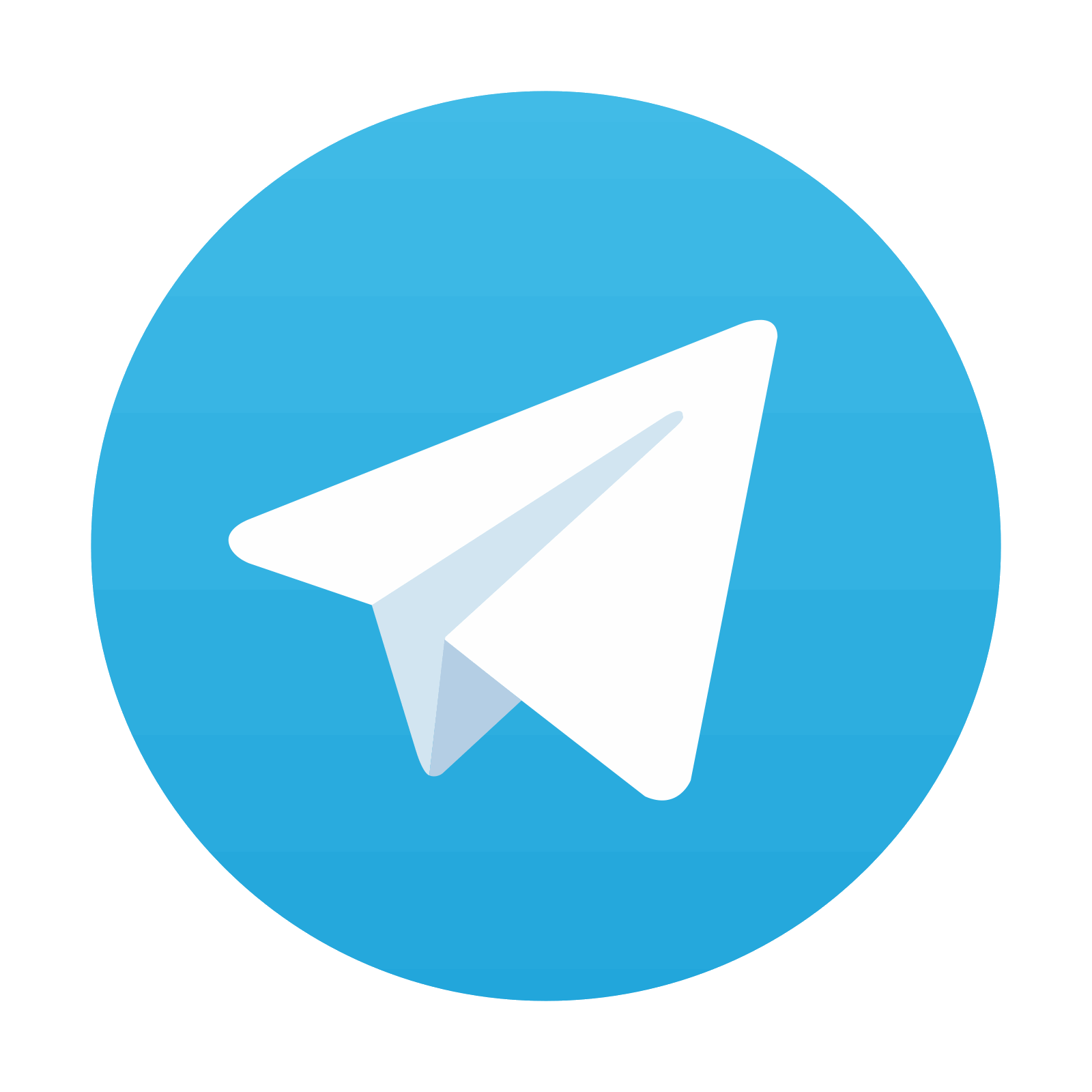
Stay updated, free articles. Join our Telegram channel
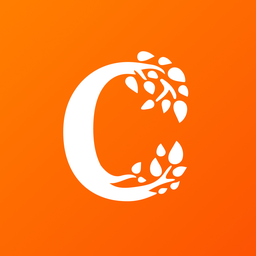
Full access? Get Clinical Tree
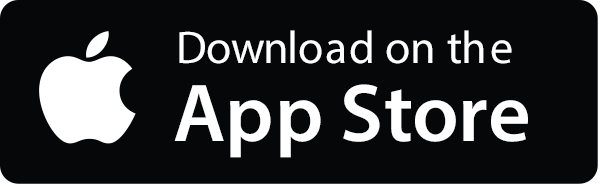
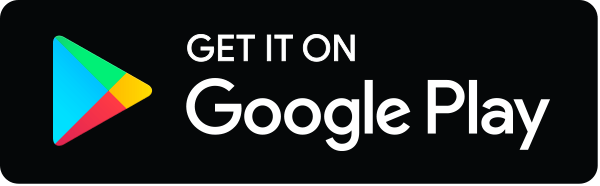