No.
Type of malignancy
Percentage (%)
0–14a
0–19a
5-year survivalb
1
Leukemias
30
51.6
47.1
80.7
2
Brain and CNS tumors
20
42.2
43.1
72.1
3
lymphomas
14
16.6
25.1
90.0
4
Soft tissue sarcomas
7
10.9
12.4
71.0
5
SNS tumors
7
10.2
7.8
75.4
6
Renal tumors
6
7.8
6.3
88.8
7
Osseous tumors
5
7.3
9.2
69.0
8
Carcinomas
3
6.7
17.1
92.7
9
Germ cell tumors
3
5.7
11.9
91.2
10
Retinoblastomas
3
4.1
3.1
97.8
11
Hepatic tumors
1
2.5
2.2
68.2
12
Others
1
0.5
0.5
The overall incidence of pediatric malignancy is increasing and has escalated from 0.5 % to approximately 1 % over the past 30 years with most of this increase having occurred in carcinoma, lymphoma, and germ cell tumors [4]. The reasons for this increase are not understood, and the pessimistic natural history of most pediatric cancers weighs against improvements in tracking and documentation of statistics as the sole cause.
Nuclear medicine and specifically positron emission tomography (PET) imaging have played a major role in the improved detection and staging of malignancy [6]. Thus PET/CT imaging is of pivotal importance in all pediatric cancer centers for most malignancies. A wealth of new PET tracers is on the horizon which promises to significantly expand the molecular imaging toolbox [7–9]. (See Chap. 17 for more details about new emerging PET tracers.)
21.2 Functional Versus Anatomic Imaging in Pediatric Malignancy
Although ultrasound is widely used in the initial detection of pediatric malignancy, CT and MR imaging remain the standard of care for the initial staging of most tumors, with the possible exception of leukemia as discussed below. As opposed to “emission tomography” which is common in nuclear medicine, computed tomography is a form of “transmission tomography” relying upon X-rays with energies generally between 60 and 140 keV depending primarily on the body habitus of the patient. As an X-ray imaging modality, CT images the spatial distribution of density, which is often significantly altered by malignancy. In addition, iodinated contrast enables the assessment of both the vascularity and, generally in a nonquantitative fashion, capillary permeability of structures under examination. 4D CT, which can potentially rigorously calculate perfusion and capillary leak in malignancy [10], is seldom used in the pediatric population due to the implicit relatively high radiation exposure and the short time over which that dose is applied. Where iodinated contrast is employed, the tube energy is often set to approximate the 32 keV K-edge of iodine [11] to maximize visibility of the agent as closely as possible while maintaining acceptable image quality. Dual energy CT, an old technique with fairly recent implementation in clinical practice, enables multi-element decomposition and can potentially supplement structural imaging with “iodine maps” which may offer improved routine assessment of these functional parameters at routine dose [12]. In CT, because the acquisition is so fast, problems such as patient motion or a mistimed contrast bolus which are common in pediatric imaging can only be corrected by repeat scanning which entails a second radiation dose. Low-dose CT is however extensively used in nuclear imaging for anatomic localization and attenuation correction and being an attenuation-based modality carries a technical advantage over MRI in this regard [13].
Conventional MR images the spatial distribution of hydrogen in water molecules using a hydrogen coil and strong magnetic fields. Unlike CT images, which can be acquired in seconds, a complete MRI requires several minutes to sometimes over an hour to acquire depending on the size of the imaging field, number and type of sequences chosen, and whether gadolinium-enhanced images are required. Thus sedation is often necessary in children, in our experience, under the age of 6 even with a skilled technologist. Although exquisite contrast resolution is available in MRI (unlike CT which offers better spatial resolution), MRI does not image tissue density directly and so carries limitations in terms of attenuation correction of nuclear images including PET. These limitations can potentially be overcome with appropriate software [14]. MRI is capable of high-end functional imaging including perfusion imaging, the imaging of molecular motion, intravascular flow assessment, and blood-oxygen-level-dependent (BOLD) imaging and offers numerous extensively described though more experimental techniques such as arterial spin labeling to assess perfusion without contrast, as well as thermometry to assess metabolic activity in the form of heat. It is important to note however that from a clinical point of view, none of these advanced tools has been shown to outperform PET/CT imaging clinically for the staging of disease or assessment of treatment response. Despite the power of MRI particularly in the brain, PET has remained a useful tool in addition to MRI to assess brain tumors for histologic grade, response to therapy, and recurrence [15–17]. In addition, because MRI is affected by signal dropout next to air, metal, or dense calcium, MRI is limited to assess the lung parenchyma, mucosal interfaces, or bone/tissue adjacent to orthopedic hardware [18–20].
Conversely nuclear imaging, especially PET when coupled with CT, excels in these technically challenging situations. In addition, PET images which are limited by problems such as motion artifact can often simply be reacquired without additional dose to the patient. All imaging modalities including CT, MRI, conventional nuclear imaging, and PET should be regarded as complimentary tools in a well-equipped imaging department, each with their own strengths.
21.3 Special Considerations in the Pediatric Population
Obtaining high-quality studies in children is both challenging and rewarding. It is important to understand that in pediatric nuclear medicine, the staff is working with both frightened child and anxious parents. Careful planning (including a flexible scheduling), communication appropriate for the child’s stage of development, appropriate injection techniques, paying attention to the imaging environment (including the use of immobilization devices or safety restraints, distraction techniques, and the possibility of sedation when necessary), and a friendly atmosphere are the key factors to deal with children and their parents. In general, it takes about twice as long to complete a procedure on a pediatric patient as on an adult.
21.3.1 Radiation Dose
Although the oncogenic potential of ionizing radiation is a function of age, with younger patients being at higher risk than older patients, the likelihood of secondary malignancy arising as a result of diagnostic medical radiation exposure, even in children, is so low as to be statistically difficult to calculate [21]. Studies published to date claiming an association between medical radiation and cancer are influenced by controversial statistical assumptions or selection bias [6].
Studies assuming a linear no-threshold model of radiation injury deemed to be a conservative view by the International Commission on Radiological Protection and back-projecting atomic bomb and nuclear accident cancer risks are deemed by some authors as inappropriate [6]. These studies are contradicted by many years of high-dose I131 therapy data for hyperthyroidism which has failed to demonstrate any definite increase in cancer risk [22, 23].
Two principles can however be universally agreed upon:
- 1.
Test only when truly medically indicated.
- 2.
When testing, use as low a radiation dose as is reasonably achievable (ALARA).
ALARA applies to technologists and physicians as much as it applies to patients [24].
Although children are more radiosensitive than adults [25], nuclear medicine tracers have an advantage over X-ray imaging techniques such as CT in that the dose is applied over a longer duration [26]. Double-stranded DNA breaks, which are repaired over 1–4 h at diagnostic doses, may not compound with each other as readily in any given cell if a patient is radiated over hours (i.e., 18F-FDG PET), days (i.e., Tc 99m), or weeks (i.e., Ga 67) for any given cumulative dose in mSv [27].
Shorter half-lives result in decreased cumulative dose. It is preferable for diagnostic purposes to avoid isotopes which emit beta or alpha particles, and as such I123 is strongly favored over I131 for scintigraphic applications. PET imaging in general employs shorter half-life tracers than conventional nuclear medicine imaging and benefits, on the whole, from a corresponding dose reduction, particularly where many of the on-site cyclotrons produced short half-life isotopes are concerned. PET dosimetry is discussed in Chap. 3.
An important caveat to the above however is in special populations, for example, children with known mutations inhibiting proper DNA repair. A classic example is retinoblastoma, where patients are so radiosensitive as to avoid sun and X-ray exposure as well as other sources of oxidative injury [28, 29]. In adults, the classic example is BRCA mutation, which is likely associated with increased radiosensitivity [30, 31]. Such special populations do become a major issue in specialized tertiary and quaternary pediatric hospitals. In these patients, there may be particularly aggressive application of ALARA, and MRI with sedation may in some instances be preferable to ionizing radiation exposure.
In 2015, it is no longer reasonable to assume that iodinated contrast media and gadolinium-based contrast agents do not produce oxidative DNA injury [32]. These agents produce the same double-stranded DNA injury as does radiation exposure, alcohol consumption [33], toxin exposure including smoking, and excessive exercise [34], all of which are discouraged in children and young adults.
The pediatric injected dose are usually calculated from the adult dose based on different formulas adjusting for weight ((body mass (kg) × adult dose)/70 kg), body surface area ((BSA (m2) × adult dose)/1.73 m2), age (Webster’s formula; (age (years) + 7) × (adult dose)/(age (years) + 1)), or the European Association of Nuclear Medicine (EANM) Paediatric Dose Card [35–37]. With the new technology and improved instrumentation, the optimum doses for children should be changed. This was addressed in the new North American consensus guidelines published in 2010 [38]. However, the selection of the appropriate dose depends on the patient population, choice of equipment, specific requirements of the clinical protocols, and the physician’s judgment [35]. Thus, deviation from the administered activities listed in the consensus guidelines can be considered appropriate when clinically indicated [35].
21.3.2 Sedation
Pharmacologic sedation can be kept to a minimum with adequate patient preparation, patient and parent education, as well as technologists that are experienced in the care of children. When absolutely necessary, the most commonly used drugs include midazolam, oral chloral hydrate, and pentobarbital [39, 40]. Patients > 15 kg with developmental delay are also most commonly sedated with either midazolam or pentobarbital [39, 40]. Pentobarbital, as all barbiturates, is contraindicated in patients with porphyria. Seizure patients subjected to pentobarbital may require dose adjustments or tapering of dose [40].
In addition to its sedative and anxiolytic effects, benzodiazepines such as midazolam have useful amnestic effect which can be particularly helpful if a patient must be exposed to multiple repeat tests in an imaging department [40]. In general each center will have its own guidelines on the sedation of children. For reference, the American Academy of Pediatrics (AAP) also publishes useful guidelines which guide best practices. Institutional protocols should be developed in collaboration with anesthesiology and include the availability of reversal drugs [39]. The continuing support and availability of anesthesiology is of immense benefit to a nuclear medicine department specializing in the care of children.
Patients with risk factors for anesthesia, including but not limited to airway obstruction, snoring, cardiorespiratory illness of any kind, asthma, intracranial pressure abnormality, or altered neck biomechanics, would benefit the most from real-time monitoring by a qualified anesthetist. Risks such as malignant hyperthermia should also be considered [40]. Playing with the patient; providing a pacifier, bottle, or (preferably non attenuating) toy; the use of distractors including nap time and room decorations; the availability of experienced and qualified technologists; and booking adequate time for imaging of difficult cases will significantly reduce the need for sedation in an imaging department.
21.3.3 Special Considerations in 18F-FDG PET/CT
The use of PET/CT was less frequent than adults for several reasons, including the concern about the radiation dose, less common frequency of children malignancy, and the lack of availability of PET/CT in pediatric centers. However, the usefulness of PET/CT in pediatric malignancy has been shown in many studies during the last decade. Although the basis of PET/CT in children is the same as adult, there are some physiologic variation in 18F-FDG biodistribution and potential pitfalls in pediatric population which are needed to be addressed for correct interpretation of the images. Some of these issues are discussed in this section.
21.3.4 Isotopes and Radiopharmaceuticals
PET isotopes are generally cyclotron produced and have shorter half-lives than their conventional nuclear medicine counterparts. Fluorine 18 (F18), with a half-life of 110 min, is among the longer lived PET tracers. The most common F18 tracer is fluorodeoxyglucose (18F-FDG). 18F-FDG has found numerous applications in the assessment and staging of cancers throughout the body, assessing response to treatment, and is used widely in the assessment of organ metabolism particularly in the heart and brain.
F18′s half-life is long enough to permit off-site production and compounding into a radiopharmaceutical of interest. Other commonly used PET isotopes include N13 (half-life ~10 min) and O15 (half-life ~2 min) which are used in N13 ammonia and oxygen 15 water studies, respectively. The half-life of these isotopes is so short that they necessitate production in an on-site cyclotron. Such cyclotrons have dropped dramatically in price in recent years. Negative ion cyclotrons are entirely self-shielded and have a small footprint in an imaging department. On-site compounding and synthesis of PET tracers can be semiautomated but does usually require dedicated personnel including, ideally, a radiopharmacist.
21.3.5 Protocols for Fluorodeoxyglucose PET
As in adult, reviewing the history, clinical indication, history of diabetes, fasting states, recent infection, patient ability to lie still during the acquisition, and the need for sedation should be reviewed before the study [41]. Patients are instructed to fast and not consume beverages, except for water, for at least 4–6 h before the administration of 18F-FDG to decrease physiologic glucose levels and to reduce serum insulin levels to near basal levels. Insulin levels greatly affect the biodistribution of 18F-FDG, and where 18F-FDG is used, care should be taken to ensure that a patient has not eaten in the 4–6 h preceding a study. Blood glucose should be checked before the administration of 18F-FDG and should be in the range of approximately 3–11 μmol/mL. Diabetic children pose a special problem and may, if they are significantly hyperglycemic, administer a weight-based sliding scale dose of insulin and wait at least an hour prior to injection. It would be better to rebook the case if possible to a day when euglycemia is achieved. Weight, height, and blood glucose concentrations are recorded for all patients.
Image acquisition for the whole body PET scan usually starts approximately 60–90 min after injection of 5.18 MBq/kg (0.14 mCi/kg) 18F-FDG, at doses ranging from 37 MBq (1 mCi) to 370 MBq (10 mCi). Patients were imaged from skull base to mid-thighs (approximately 3 min per bed position). The number of bed positions depends on the size of the patient, and it is usually less than 7 bed positions in small children and 7–10 bed positions for adolescents. In general, the arms are elevated over the head to avoid beam-hardening artifacts over the torso [41]. However, for optimal imaging of the head and neck, the arms should be positioned along the side [41]. The patient should void before the acquisition of the images to limit the radiation dose to the kidneys and bladder and also to be able to lie still on the bed for the acquisition time. Metallic objects and any other unnecessary objects should be removed from the patient whenever possible [41].
Dual-time point 18F-FDG PET imaging usually consists of initial imaging at 60 min after tracer injection and then reimaging later at 2–4 h postinjection. Dual-time point image is based on the premise that 18F-FDG uptake in inflammatory cells usually washes out, while 18F-FDG tends to be retained in malignant cells [42, 43]. Dual-time protocols have been used in pediatric population but for logistic reasons are not frequently applied in practice [44].
In children and adolescents and particularly when the weather is cold, it can be helpful to provide an ambient room for approximately 1 hour prior to injection of 18F-FDG to decrease metabolism in brown fat [45]. Patients who had recently undergone diagnostic CT scans are imaged using a low-dose helical CT scan (in our protocol: 5 mm/slice, 90 kV; 20 and 30 mA for patients weighing < 30 and ≥ 30 kg, respectively) prior to the PET scan for attenuation correction and anatomic localization. Diagnostic CT scans were obtained when clinically indicated and when patients do not have a recent CT scan. In those cases, the attenuation correction is calculated based on the correlative diagnostic CT images (in our protocol: 5 mm/slice, 120 kV, and a weight-based range for the mA, with dose modulation).
21.3.6 F18-FDG PET Normal Variants and Pitfalls in Children
The normal distribution of 18F-FDG uptake in children is unique and may differ from that in adults. A number of physiologic variants are commonly seen, including normal physiologic activity in the head and neck, heart, breast, thymus, liver, spleen, gastrointestinal tract, genital system, urinary collecting system, bone marrow, muscles, and brown adipose tissue [46]. Benign lesions with increased 18F-FDG activity are also frequently seen and can be misinterpreted as malignancies [46]. Other parameters such as the standardized uptake value (SUV) can also be different in pediatric population.
21.3.7 Standardized Uptake Values
The standardized uptake value (SUV) is a measurement unique to PET and is defined as attenuation-corrected activity in a region of interest divided by decay-corrected injected activity divided by body mass [47, 48]. It is also important to note that activity in a region of interest does change somewhat with different devices and reconstruction algorithms [49]. Still, standardized uptake value is the key semiquantitative parameter on which PET quantification is based and can be presumed to be reasonably reliable when patients are reimaged on the same device with the same dose of tracer over a time frame such that body weight is reasonably consistent.
SUVmax, or the largest SUV value in an ROI, is often chosen due to its reproducibility between readers (i.e., cancers are generally hotter on PET toward their center of mass, due to both cellular density and partial volume effects). Changes in SUV or SUVmax can indicate the aggressiveness of disease or response to therapy. In general, cancer responding to therapy will show a decrease in SUVmax, with the exception of radiation treatment of tumor which will often go through a short-term hypermetabolic phase followed by a gradual decline (see below). Measurement of SUV in small ROIs can be made less reliable by partial volume effects [50] (see Chap. 15 for further details).
It is important to recognize that fat has a relatively low metabolic rate, and so some authors prefer to normalize to lean body mass or body surface area in the SUV calculation, particularly in obese patients, where normalized to body weight SUV can be artifactually overestimated in non-adipose tissue [48]. The percentage of fat is changing in pediatric patients from 11 % in the newborn to about 26 % during the following 5 months and then decreases gradually until 12 months of age [51]. After that the percentage of fat depends on many factors including diet, physical activity, and genetics [51]. Thus, pediatric SUV normalized to body weight is not exactly the same as that of adults. Moreover, the clinical significance of SUV values in different pathologies and normal versus suspected malignancy reference numbers in children cannot be simply extrapolated from the adult values and should be interpreted cautiously. The optimal method for calculating SUV in children may be different from that used in adults due to the body changes and growth that take place during childhood. Therefore, it has been suggested that, in pediatric patients, SUV calculated on the basis of body surface area would serve as a better metabolic activity marker than would SUV calculated on the basis of body weight.
21.3.8 Thymus
Thymic tissue persists in children and often persists into early adulthood. Thymus is often mildly to moderately 18F-FDG avid on PET and can be mistaken for mediastinal tumor (Fig. 21.1). Thickness of the thymus gland, measured perpendicular to a lobe, should be less than 1.8 cm in children under twenty and 1.3 cm in older patients [52, 53]. The shape of the thymus is quadrilateral during childhood and triangular during adolescence. Morphologic features such as thickness of the gland and its homogeneity are used on either diagnostic or low-dose localizer CT to determine the presence of disease [53].
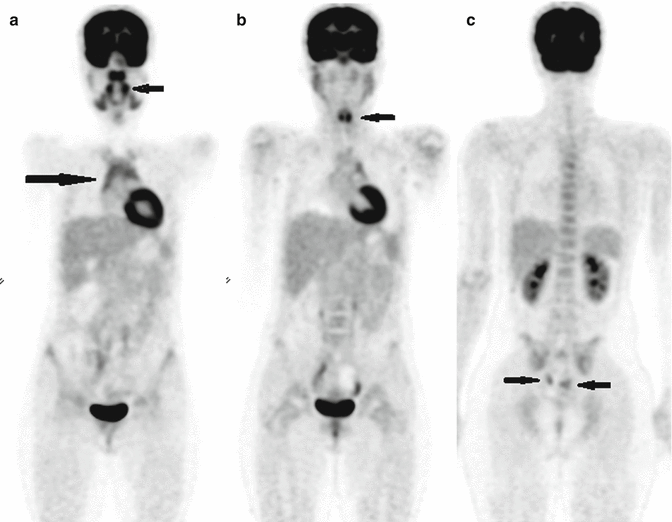
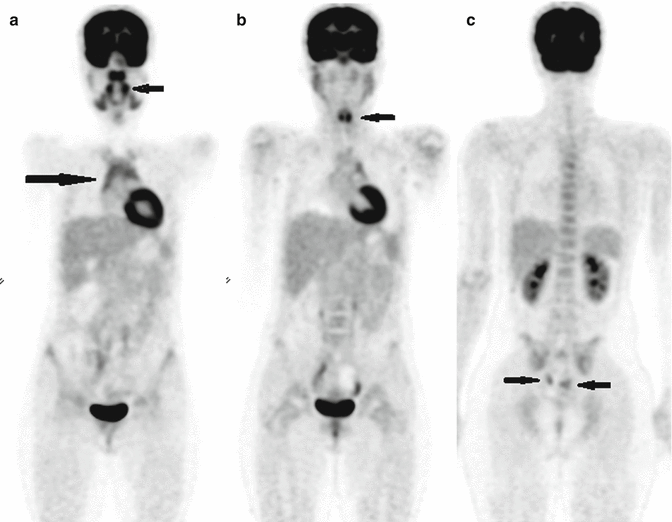
Fig. 21.1
Physiologic activity in a 13-year-old female. (a) Physiologic activity in the palatine and lingual (small arrow) tonsils and normal uptake in the thymus (long arrow). (b) Physiologic activity in the vocal cords or muscles in the vocal cord region (arrow). (c) Normal uptake of the bone marrow. Foci of activity in the pelvic region due to follicular activity of ovaries (arrows)
Thymus uptake of 18F-FDG can increase following chemotherapy which is a frequent consequence of chemotherapy, especially in young patients undergoing chemotherapy for lymphoma, leukemia, or testicular cancer. The reasons for this hyperplasia are debated. An important function of the thymus is to “train” lymphocytes toward adaptive immunity. Chemotherapy is associated with decreased white counts and thymus atrophy. Steroids, frequently concomitant with chemotherapy for the treatment of lymphoma, can also induce thymus atrophy which then “rebounds” with subsequent marrow hyperplasia [54].
It can be challenging to differentiate benign from malignant thymus 18F-FDG activity. In general, homogeneous thymic uptake at post-therapy 18F-FDG PET and the absence of uptake at pretherapy 18F-FDG PET indicate post-therapy thymic hyperplasia. To some extent the SUV of thymus tissue can guide decisions. Brink et al. reported a mean SUV of 2.8 and max of 3.8 in thymic hyperplasia [54]. Ferdinand et al. indicates that thymic SUV > 4 warrants further investigation to exclude malignancy [55].
Thymic carcinoma is a rare malignancy in children and in general is associated with significantly increased 18F-FDG uptake. Sasaki et al. reported SUV of 7.2 ± 2.9 in thymus carcinoma, which is in turn significantly higher than activity found in invasive thymoma (3.8 ± 1.3) and noninvasive thymoma (3 ± 1) [56]. Thymoma can therefore not be reliably differentiated from thymus hyperplasia. Morphologic features on CT are helpful in these cases to differentiate benign change from malignancy.
21.3.9 Tonsils
The tonsils are very metabolically active in children and can demonstrate significant 18F-FDG avidity on PET scan (Fig. 21.1). The palatine and lingual tonsils, in part because of their size, are particularly avid. Upper respiratory tract infections can cause tonsillar hypertrophy and increased thymus metabolism. The symmetry of tonsil activity is an important means of differentiating benign from malignant processes [57]. Lymphoma in the head and neck, oropharyngeal malignancy, and posttransplant lymphoproliferative disorder (PTLD) are all associated with increased 18F-FDG activity and can mimic or be difficult to differentiate from normal functioning tonsillar tissue. Comparison to any prior 18F-FDG PET imaging and correlation to CT, ultrasound, or MR imaging can be helpful in the assessment of challenging cases.
21.3.10 Marrow Hyperplasia
Marrow suppression is a frequent complication of chemotherapy with or without colony-stimulating factor administration and can also result from tumor infiltration of bone marrow. After treatment, most often by chemotherapy or steroids, suppressed marrow can subsequently rebound and demonstrate diffuse hypermetabolism, with increased activity on 18F-FDG PET that can be confused for diffuse malignant change [58–60]. Anemia, including thalassemia, medications such as interferon can also cause diffuse increased bone activity and represent an important cause of false-positive findings [61, 62]. Treatment with hematopoietic cytokines such as granulocyte colony-stimulating factor (CSF), hematopoietic growth factor, or erythropoietin can also produce diffuse skeletal 18F-FDG accumulation. Increased activity can persist for up to 3 weeks after the discontinuation of granulocyte CSF treatment. Increased activity in the spleen is also frequently noted in association with increased marrow activity (Fig. 21.2). Normal bone marrow is mildly 18F-FDG avid that is less intense than liver activity (Fig. 21.1).
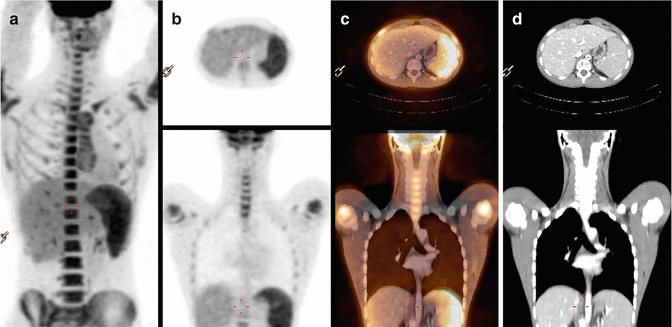
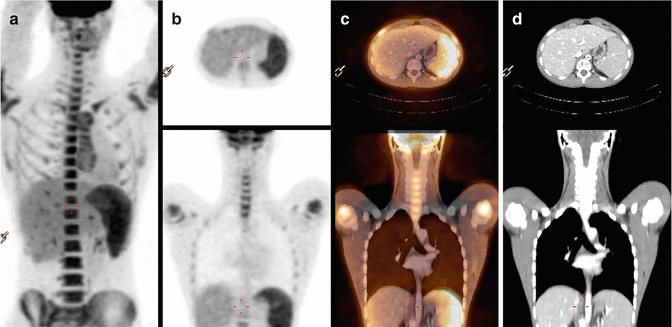
Fig. 21.2
18F-FDG PET/CT in a 17-year-old patient with lymphoma after two cycles of chemotherapy and one day after injection of GCSF. (a) Maximum intensity projection (MIP), (b) Axial and coronal PET, (c) Axial- and coronal-fused PET/CT, and (d) CT scan showed marked improvement of the activity previously visualized in the mediastinum (not shown here). Increased activity in the spleen and bone marrow is due to GCSF effect
21.3.11 Brown Fat
Physiologic high uptake from activated adrenergic innervation of adipocytes is a normal variant especially in children. This brown fat, rich in mitochondria, is more common in children than adults and is typically found in the neck, supraclavicular regions, axillae, mediastinum, and paravertebral and perinephric regions. The distribution of brown fat is usually symmetric. However, focal and asymmetric uptake can occur in the neck or mediastinum, leading to false-positive results.
Brown fat because of its 18F-FDG avidity can present a considerable problem in the interpretation of 18F-FDG PET studies (Fig. 21.3). The most practical way to control brown fat activity is to control the temperature of the patient before injection and in the period between injection and image acquisition [45, 63]. Some authors have also used medication, most commonly propranolol and diazepam, to decrease activity in brown fat [63].
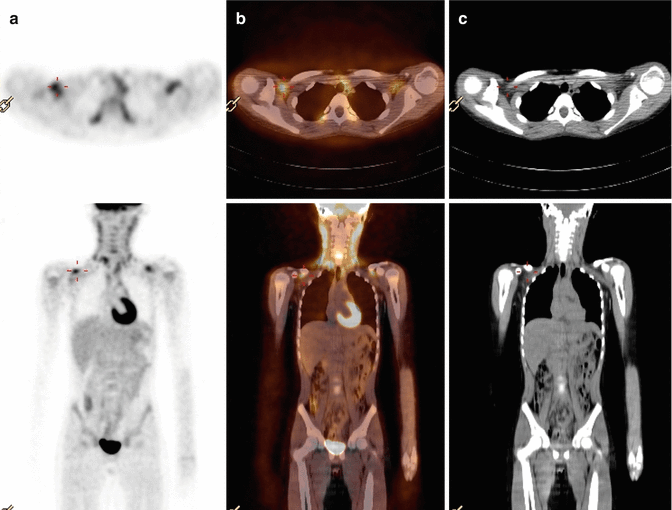
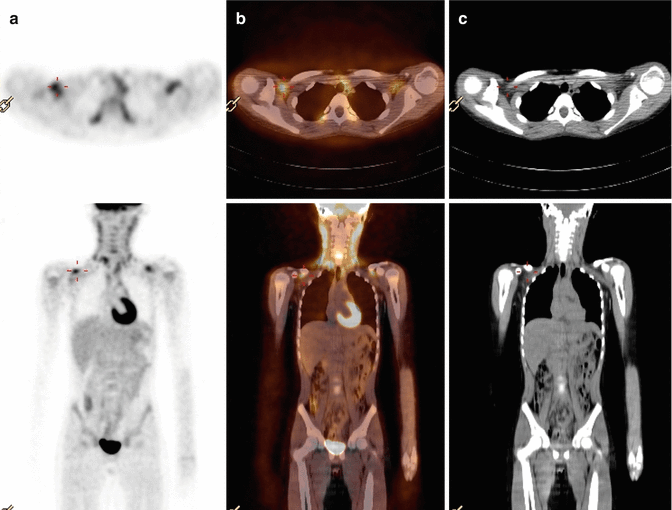
Fig. 21.3
Axial and coronal PET (a), fused PET/CT (b), and CT (c) of 18F-FDG PET/CT in a 12-year-old boy diagnosed with large B-cell lymphoma 3 years before, referred for evaluation of recurrence. Multiple foci of FDG activity are noted in the neck, supraclavicular region, and chest bilaterally corresponding to the hypodense regions on CT due to brown fat uptake. The patient had positive neck lymph nodes, an FDG-avid lesion in the right lung, and lumbar spine involvement which were not shown here
Fusion imaging with CT enables to differentiate between 18F-FDG uptake corresponding to fat-attenuation tissue at CT and uptake from pathologic causes.
21.3.12 Posttreatment Change
It is generally recommended to wait at least 4–6 weeks after surgery and at least 1–2 weeks after biopsy prior to performing 18F-FDG PET [64]. A shorter interval than this has been associated with physiologic increased uptake in healing tissues which could be mistaken for a false positive. It is important for institutions to develop a practice pattern in conjunction with the treating physician.
Radiation therapy is associated with inflammation and the recruitment of white blood cells which are intensely 18F-FDG avid. Thus, tumors subjected to radiotherapy can demonstrate marked increased 18F-FDG uptake which can persist for weeks. It is ideal to wait 6 weeks after radiation therapy prior to PET imaging to assess response [64]. Reduced bone marrow 18F-FDG uptake can be noted several months after external beam radiation therapy. This phenomenon has been attributed to the replacement of bone marrow by fatty tissue.
21.3.13 Other Pitfalls
If children are significantly active before 18F-FDG administration or in the interval between 18F-FDG administration and imaging, uptake in metabolically active muscles may limit assessment. It is best for patients undergoing 18F-FDG PET imaging to avoid strenuous exercise or vigorous sports in the days which precede imaging. Chewing of gum or sucking pacifier after 18F-FDG injection can cause symmetric intense uptake in the masseter muscles. Uptake in the diaphragm, the crura of the diaphragm, and the intercostal muscles can be detected in children who have been crying during the uptake phase.
A common source of a false negative in 18F-FDG PET scan is altered biodistribution caused by improper patient preparation. Usually this is due to increased circulating insulin and characterized by decreased uptake in tumor, decreased uptake in the brain, and increased uptake in peripheral skeletal muscle. Repeating the exam may be indicated in such a situation [72].
A second common cause of a false negative is the collision of tumor activity with the normal biodistribution of activity and can be a problem particularly in the genitourinary system on 18F-FDG PET as 18F-FDG is cleared by the kidneys [72]. Visibility of cancers in the genitourinary tract can be limited on 18F-FDG PET. If possible and age appropriate, patients should empty the bladder prior to 18F-FDG PET image acquisition.
21.3.14 Other Radiopharmaceuticals
Studies on the use of other PET tracers beyond 18F-FDG in pediatric population are limited. 18F-sodium fluoride (18F-NaF) has been extensively used in adult for detection of bone metastases in different tumors and can also potentially be useful in pediatric patients [65, 66]. Accumulation of 18F-NaF in the skeletal system is similar to that of 99mTC-MDP based on local blood flow and osteoblastic activity. However, the protein binding is lower allowing for earlier images than conventional bone scan. The extraction of 18F-NaF is also higher than 99mTC-MDP. Thus the image contrast is better with 18F-NaF. PET images also provide better spatial resolution than conventional gamma cameras. However, the radiation dose is higher than the bone scan. The recommended dose of 18F-NaF is approximately 2.2 MBq/kg, with a minimum of 11.1 MBq and a maximum of 148 MBq [67]. Imaging starts 45–60 min after the radiotracer administration. The biodistribution of the tracer is similar to the 99mTC-MDP and depends on the patient’s age with more activity in the growth plates.
3′-Deoxy-3′-[F18]-fluorothymidine (18F-FLT) FLT is an analog of thymidine and the uptake reflects cellular proliferation. Thus increased activity with 18F-FLT is more suggestive of tumor proliferation than inflammation. FLT is retained in proliferating tissues and malignant tumors through the activity of thymidine kinase 1 (TK1), an enzyme that is highly expressed during the DNA synthesis phase of the cell cycle [68]. TK1 phosphorylates FLT to form negatively charged FLT-monophosphates which are impermeable to the cell membrane. Since most tumor cells have a much higher TK1 activity than normal cells, the intracellular trapping of FLT and accumulation of radioactivity occurs [68]. Current published literature have demonstrated the feasibility of using FLT PET imaging in patients with lung cancer [69–72], gastrointestinal cancer [73, 74], melanoma [75], lymphoma [76, 77], breast cancer [78, 79], soft tissue sarcomas [80], as well as primary malignant brain tumors in adults and children [81–85]. 18F-FLT has been safely administered to children between the ages of 2–13 years with no observable adverse effects [81–85]. 18F-FLT minimally crosses intact blood–brain barrier [86, 87], thus the high background activity which is typically visualized with 18F-FDG is not seen using 18F-FLT. Published literature related to the use of 18F-FLT PET in the evaluation of CNS tumors in the pediatric population is limited and restricted only to studies involving children with glioblastoma [81–85]. The recommended dose is 5.18 MBq/kg (0.14 mCi/kg) using a minimum dose of 37 MBq (1 mCi) up to a maximum of 370 MBq (10 mCi). Imaging is usually performed 45–60 min after the radiotracer administration.
Tumors have varying degrees of hypoxia. Tumor hypoxia may be related to chaotic vasculature in some tumors or rapid growth in others. It is usually associated with increase angiogenesis and tumor aggressiveness [88, 89]. Studies have shown that tumor hypoxia increases the risk of metastases and decreases the sensitivity to chemo/radiation therapy [90–93]. This may be due to the aggressiveness of the tumor or abnormal vasculature that may diminish delivery of anticancer therapy. Using hypoxia-selective cytotoxins may increase the effect of chemo/radiation therapy [94–97]. Tumor hypoxia has been also reported to be associated with increased risk of recurrence and a poorer prognosis [89, 98]. Detection of hypoxic components of tumors is possible with histologic evaluation. However, due to the patient situation, tumor type, location, etc., surgical resection of tumors is not recommended in all malignancies. Moreover, in some instances, information of hypoxic component of tumor is needed before the surgical resection (e.g., before neoadjuvant treatment in osteosarcoma). Tissue biopsy is inaccurate in these situations since it may not reflect the whole tumor. Imaging is a surrogate marker of histological assessment to detect these hypoxic components as it is noninvasive and repeatable and represents the whole tumor. 1-α-D-(5-deoxy-5-[18 F]-fluoroarabinofuranosyl)-2-nitroimidazole (18F-FAZA) is a 2-nitroimidazole-based molecule that undergoes reductive metabolism under hypoxic conditions, producing reactive intermediates that bind to intracellular macromolecules. Studies in adult population and different tumors have shown that 18F-FAZA is a promising radiotracer for detection of hypoxic components in tumors [96, 99]. The recommended dose is 5.2 MBq/kg (0.14 mCi/kg) using a minimum dose of 37 MBq (1 mCi) up to a maximum of 370 MBq (10 mCi). Imaging is usually performed 2–3 h after the radiotracer administration (hypoxia tracers are reviewed in Chap. 17).
11C-Methionine (11C-METH) is an amino acid PET tracer mainly used in brain imaging tumors. The uptake in brain tumor is probably related to passive diffusion from the altered blood–brain barrier and active uptake by the tumor due to increased amino acid metabolism. Similar to the 18F-FLT, the background activity is very minimal with 11C-METH, allowing detection of small viable brain tumors. The pediatric studies were limited; however, they showed a higher sensitivity of 11C-METH for the detection of viable brain tumor than that of 18F-FDG [100, 101]. The pediatric dose and protocol are not well established. In general, due to the short half-life of C11, imaging will start 20 min after the administration of 5.5 MBq/kg of the radiotracer [101].
68Ga-DOTA-conjugated peptides [68Ga-DOTA0-Tyr3]octreotide (68Ga-DOTATOC, 68Ga-edotreotide), [68Ga-DOTA0-1NaI3]octreotide (68GaDOTA-NOC), and [68Ga-DOTA0 -Tyr3]octreotate (68GaDOTA-TATE) have been used to detect somatostatin receptor-positive tumors. Somatostatin receptors are positive in a variety of tumors [102]. The activity administered ranges from 100 to 200 MBq in adults [102]. The exact dose in pediatric patients is not well established; the dose in children should be reduced according to the recommendations of the EANM Paediatric Task Group [102]. Imaging is usually obtained 45–90 min after the radiotracer administration depending on the type of analog used [102].
Other radiotracers like 11C-hydroxyephedrine (HED), 11C-epinephrine, and 18-F-dihydroxyphenylalanine (DOPA) have been also used for sympathetic nervous system and neuroendocrine tumors. 11C-HED is similar to norepinephrine (NE), but unlike NE it is not metabolized and is used for sympathetic nervous tumors imaging [103]. 11C-Epinephrine has been used in pheochromocytoma and neuroblastoma [104]. 18F-DOPA has been also used in pheochromocytoma, hyperinsulinemia, and brain tumors [105].
21.4 Image Fusion
Nuclear medicine image techniques benefit strongly from attenuation correction to mitigate the effects of photon absorption by the patient. Although historically transmission attenuation correction was used for this purpose [106], the widespread dissemination and reduced cost of CT led to the coupling of PET and SPECT to low-dose CT exams for anatomic localization and correction. Attenuation, itself a function of density, is ideally corrected by the density maps produced in CT. Acquisition of CT data with PET data usually minimizes the registration problem.
Increasingly in pediatric nuclear medicine practice, where a diagnostic CT is required, PET imaging can be acquired simultaneously with diagnostic CT on a hybrid device supporting a multi-slice CT scanner [107]. PET/MRI is a more recent development which enables the simultaneous acquisition of PET and MRI data, though at considerable expense and with potentially longer acquisition times. Pediatric centers, which usually have to sedate children undergoing MRI [108], often cite both this fact and the limitations of MRI for attenuation correction as a reason not to opt for a hybrid PET/MRI device. Using multisequence MRI to perform attenuation correction reliably is an area of active investigation, and significant advances have been recently made [109–112].
21.5 Tumors of the Sympathetic Nervous System
21.5.1 Neuroblastoma
Sympathetic nervous system (SNS) tumors account for about 7 % of all pediatric malignancies. Neuroblastoma, including ganglioneuroblastoma, is the most common form of all SNS tumors in children (approximately 97 %). Neuroblastoma (NBL) is a common lesion in young children and unfortunately associated with significant mortality. NBL accounts for 20 % of malignancy in children diagnosed below the age of 1 year and is especially common during the first 3 months of infancy. NBL is the second most common, after brain tumors, solid malignancy in childhood.
The long-term survival of NBL patients remains challenging. Age remains among the most important predictors of survival in NBL patients, with presentation beyond 5 years associated with a 5-year survival of approximately 40 %. Survival in younger children, especially less than 1 year, has improved dramatically since 1975, from approximately 35–83 % today as a result of improved therapy. Aside from age, stage at diagnosis is a very important prognostic indicator, with complete resection of a local tumor and negative margins indicating a more optimistic long-term prognosis. Molecular and cytogenetic factors including DNA content, proto-oncogenes, and catecholamine synthesis are all linked to prognosis.
The etiology of neuroblastoma is unclear. The predilection of the tumor in infants has implicated genetic factors or in utero toxicity, for example, alcohol [113] or other drug exposure, as potentially playing a role in causation [114, 115]. The effects of maternal substance and/or environmental exposures on the development of neuroblastoma is however controversial [116].
Diagnosis of neuroblastoma is usually enabled by a combination of imaging findings and elevated serum or urine catecholamines. There is a spectrum of catecholamine metabolites including dopamine, homovanillic acid, and vanillylmandelic acid which can be represented in neuroblastoma patients [117].
The natural history of neuroblastoma is quite variable, with some patients demonstrating spontaneous regression and some patients demonstrating differentiation of tumor into mature nonmalignant tissue. Other NBLs demonstrate aggressive behavior despite multimodality treatment and are associated with poor outcomes.
The International Neuroblastoma Staging System (INSS) uses the distribution of disease as evidenced by imaging studies, operability, lymph node metastases, and bone marrow metastases as factors influencing prognosis (Table 21.2). The more recent International Neuroblastoma Risk Group (INRG) system describes 13 potential prognostic factors to categorize patients into four groups (5-year survival of <50 %, 50–75 %, 75–85 %, and >85 %) [118].
Table 21.2
International Neuroblastoma Staging System (INSS)
Stage | Description |
---|---|
1 | Localized tumor with complete gross excision (with or without microscopic residual disease) with no ipsilateral lymph nodes involvement |
2a | Localized tumor with incomplete gross excision and no ipsilateral lymph involvement |
2b | Localized tumor with or without complete gross excision, with ipsilateral lymph nodes involvement |
3 | Unresectable unilateral tumor crossing the midline (with or without regional lymph node involvement); or localized unilateral tumor with contralateral regional lymph node involvement; or midline tumor with bilateral extension which is unresectable or with positive lymph nodes |
4 | Any primary tumor with involvement of distant lymph nodes, bone, bone marrow, liver, skin, and/or other organs (except as defined for stage 4S) |
4s | Localized primary tumor (as defined for stage 1, 2A, or 2B), with metastases limited to the skin, liver, and/or bone marrow (limited to infants 1 year of age) |
Metastases are unfortunately common at presentation, and hence multimodality imaging is important in the initial assessment of neuroblastoma [119]. Ultrasound is a frequent first test in a child with typical symptoms of abdominal mass, pain, anemia, fever, weight loss, paraneoplastic syndrome, and occasionally blindness. Ultrasound is usually followed by diagnostic CT or MRI. With positive biochemistry, scintigraphy is generally employed I123 MIBG for definitive workup [120, 121].
MIBG scintigraphy has been extensively described for the workup of NBL and remains standard of care at even quaternary pediatric oncology centers [120, 121]. MIBG has been used in prognostication and in assessment of response to therapy, with a decrease or absence of MIBG activity following therapy indicative of a good prognosis. Similarly, as bone metastases are quite common in NBL, the Tc 99m MDP bone scan has been used extensively for the assessment of osseous metastases [122]. However, in approximately 10 % of cases, NBL is not MIBG avid (Fig. 21.4).
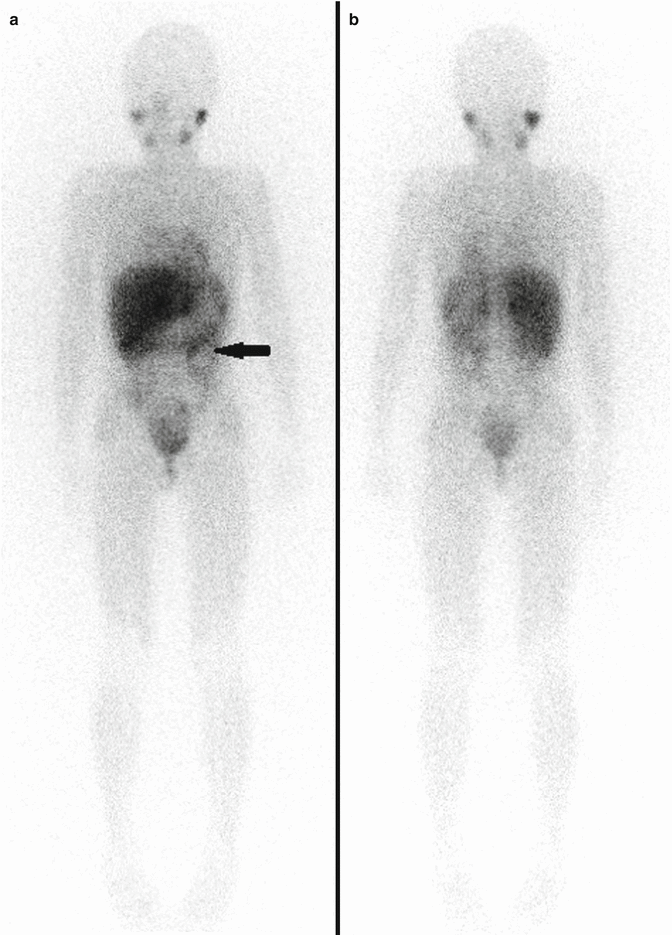
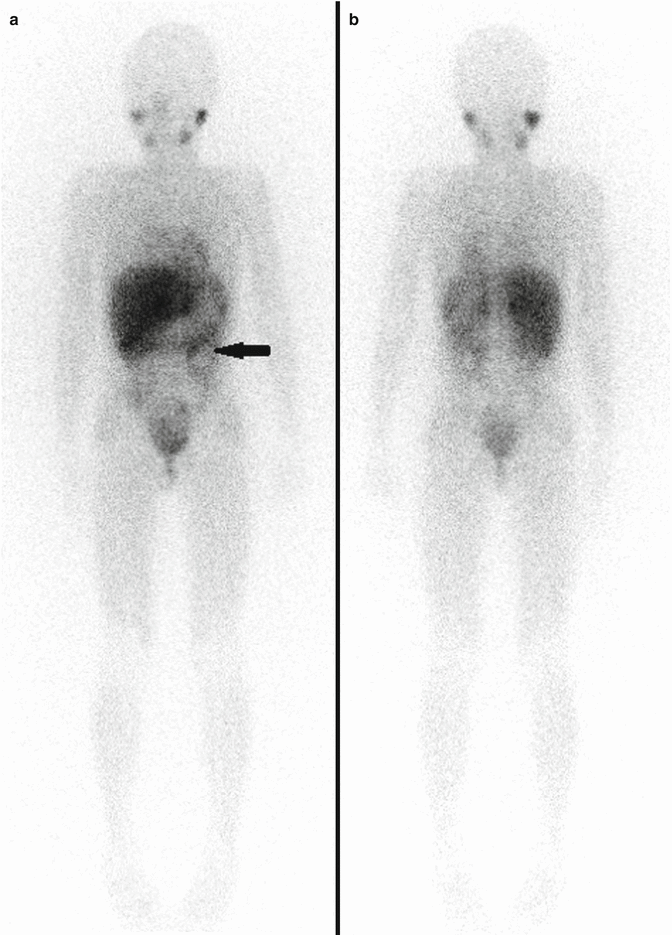
Fig. 21.4
Twelve-year-old male with neuroblastoma in the left abdomen. (a) Anterior and (b) posterior MIBG scan showed mild MIBG activity in the region of the tumor (arrow). The liver uptake was inhomogeneous with no definite focal increased activity
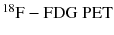
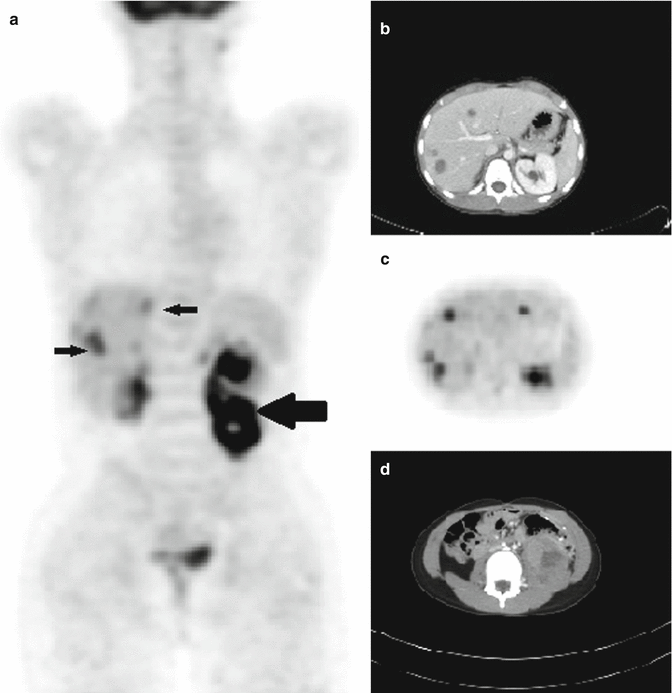
Fig. 21.5
18F-FDG PET/CT in the same patient as described in Fig. 21.3, with a left paravertebral mass with very mild MIBG uptake. (a) Coronal PET showed a heterogenous intense uptake in the left abdominal paravertebral lesion as well as multiple foci of increased activity in the liver suggestive of metastases. A superimposed infectious process in the liver could not be excluded. Biopsy proved the diagnosis of metastatic neuroblastoma. (b) Axial CT from the upper abdomen and (c) axial PET showed multiple lesions in the liver. (d) Axial CT from the abdomen shows the left abdominal paravertebral mass

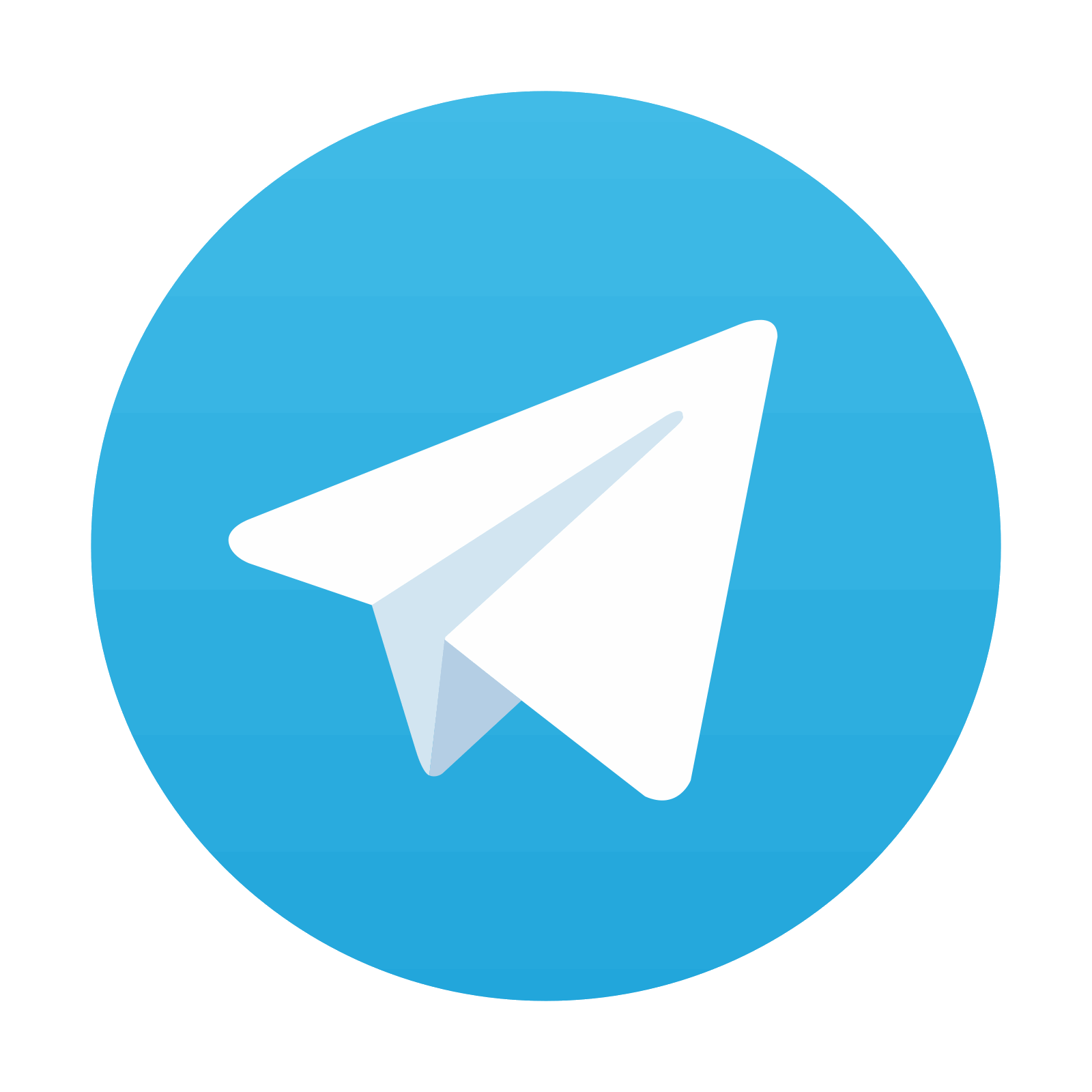
Stay updated, free articles. Join our Telegram channel
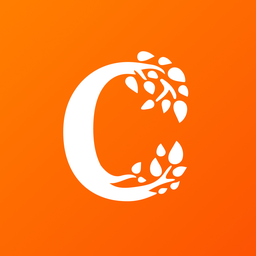
Full access? Get Clinical Tree
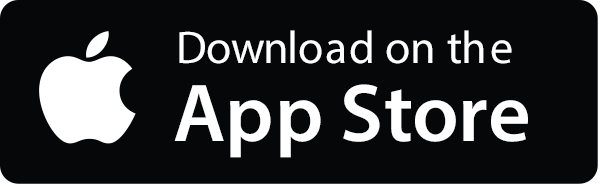
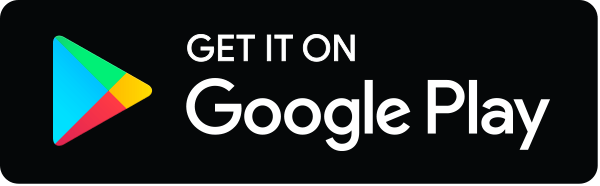