Fig. 15.1
Schematic summary of the transvascular-interstitial-transmembrane pathway in USMB-mediated drug delivery. (a) Potential mechanisms responsible for the passage across the vessels include creation of temporary gaps between vascular endothelial cells by volumetric expansion and contraction of the oscillating microbubbles. Also, cavitation of microbubbles may disrupt vascular endothelial cell integrity during violent collapse of microbubbles, creating ruptures in the vascular endothelial layer. (b) Interstitial transport of drugs/genes within the extracellular matrix may be enhanced by ultrasound radiation force. (c) USMB may induce membrane disruption and/or enhance active transport, such as endocytosis, thereby enhancing cellular permeability
Bekeredjian et al. (2007)) injected Evans blue dye (a highly charged low molecular weight marker that binds to serum albumin (about 69 kDa) to become a high molecular weight intravascular tracer protein) (Hoffmann et al. 2011; Elodie Debefve et al. 2013) and lipid microbubbles into hepatoma-bearing rats and insonated the tumors with ultrasound (1.3 MHz, mechanical index 1.6, bursting pulses every 4 cardiac cycles for 15 min). They showed an approximate five-fold higher Evans blue dye accumulation in insonated compared to non-insonated tumors. The amount of Evans blue extravasation has also been shown to be affected by both the microbubble type and the acoustic conditions. Bohmer et al. (2010)) showed that ultrasound (10,000 cycles of 1.2 MHz ultrasound pulsed at 2 MPa pressure and a pulsing rate of 0.25 Hz for 5 min) increased Evans blue extravasation by a factor of 2.3 in the presence of lipid-shelled mircobubbles, compared to a factor of 1.6 in the presence of polymer-shelled microbubbles in murine colon cancers subcutaneously established in mice. This difference likely occurred because the cavitation threshold is lower for lipid-shelled microbubbles. In the same study, two acoustic conditions were compared: Pulse lengths of 100 and 10,000 cycles. The results showed that the spatial extent of extravasation was significantly smaller for 100 cycles per pulse than for 10,000 cycles (6 ~ 9 mm vs. 18 ~ 20 mm), while other ultrasound parameters (1.2 MHz, 2 MPa pressure and pulsing rate of 0.25 Hz for 5 min) and the microbubble type (polymer-shelled microbubbles) were kept the same.
Since Evans blue dye bound to serum albumin is relatively small (7 nm) (Elodie Debefve et al. 2013) compared to many therapeutic agents, another study assessed whether USMB can also increase tumor delivery of other model drugs that are larger in size. Carlisle et al. (2013)) demonstrated that USMB (ultrasound: 0.5 MHz, 50,000 cycles pulse length, 0.5 Hz pulse repetition frequency, 1.2 MPa peak rarefactional pressure for 4 min; SonoVue® microbubbles) increased extravasation and intratumoral (i.t.) distribution of a 130-nm luciferase labeled polymer-coated adenovirus in a breast cancer mouse model. Compared with non-insonated tumor, USMB resulted in a 5-fold increase in the amount of delivered adenovirus within 100 μm of blood vessels, and an increase of 40 fold beyond 100 μm. This suggests that USMB may not only increase the amount of drug extravasation, but also enhance drug penetration in the tumor interstitium, as shown in the following section.
15.2.1.2 Interstitial Transport
The high interstitial fluid pressure in tumors can reduce the convectional transport of drugs and particles throughout the extracellular matrix and, hence, only a small population of neoplastic cells located close to blood vessels is exposed to therapeutic agents by diffusion (Bae 2009; Davies Cde et al. 2004). Application of ultrasound has been shown to facilitate drug penetration beyond the close proximity of tumor vessels, potentially through radiation force caused by ultrasound (Fig. 15.1b). Radiation force is produced by the pressure gradient caused by a momentum transfer from the wave to the attenuating media, arising either from absorption or reflection of the wave. This momentum transfer from ultrasound beam to a particle causes the transport of the particle in the direction of wave propagation. Due to higher tissue absorption at higher frequencies, the radiation force increases with increasing frequencies.
In a study performed by Eggen et al. (2013), prostate tumors established in mice were exposed to ultrasound (1 or 0.3 MHz, 13.35 W/cm2, mechanical index 2.2, 5 % duty cycle, total exposure 10 min) 24 h after the administration of liposomes. At this time point, liposomes had passively extravasated into the tumor via the enhanced permeability and retention (EPR) effect, with a very low remaining concentration in the circulation (only approximately 10 % of liposomes in the circulation 24 h after injection). Since the blood liposome concentrations at 24 h was so low, changes of liposomal tumor distribution following ultrasound application was considered to be caused by its effect on already extravasated liposomes, rather than liposomes still in circulation. The study showed that liposomes in tumors insonated with ultrasound were more scattered throughout the tumor volume and penetrated two-fold more from blood vessels compared to those in non-insonated tumors. Moreover, the penetration distance was larger when the higher frequency (1 MHz) was applied. One potential explanation for this phenomenon is that the acoustic radiation force enhances drug transport. As the ultrasound frequency increased from 0.3 to 1 MHz, the radiation force could be increased, thus facilitating the transport of particles in the interstitium. This study demonstrated that radiation force from ultrasound may propel interstitial transport of extravasated therapeutic agents within the interstitial space. The increased penetration depth may allow the therapeutic agents to act on deeper lying tumor cells, eventually improving the outcome of tumor drug therapy.
However, the penetration depth of therapeutic agents varies at different spatial locations within tumors. Eggen et al. (2014) showed that USMB had a different impact on drug delivery in the periphery versus the core of tumors. In a prostatic cancer mouse model, USMB resulted in an increased nanoparticle penetration distance of 0.5–1 nm in the tumor periphery compared to the tumor core. This phenomenon was potentially caused by the heterogeneous distribution of interstitial fluid pressure across tumors. Indeed, in other studies using subcutaneous rat breast cancer models (Boucher et al. 1990) and a subcutaneous human osteosarcoma xenograft model (Eikenes et al. 2004), the interstitial fluid pressure rose with increased distance from the tumor periphery to the core within the first 400 μm, and then plateaued afterwards. The elevated interstitial fluid pressure may hinder transport of the extravasated particles in the interstitial space, resulting in shorter penetration in the tumor core.
15.2.1.3 Transmembrane Transport of Tumor Parenchymal Cells
The process of USMB-mediated permeability enhancement of tumor cells can be induced by pore formation and/or active transport across the membrane (Fig. 15.1c).
Pore formation in tumor cells has been visualized in many in–vitro studies, such as melanoma C32 cells (Matsuo et al. 2011) and prostate cancer DU145 cells (Zhang et al. 2012) by electron microscopy. Stable and inertial cavitations have been shown to cause cell membrane displacement and disruption for improved drug delivery across cellular membranes (Taniyama et al. 2002; van Wamel et al. 2004). Pore sizes between 100 nm and a few micrometers have been reported (Schlicher et al. 2006). This implies that exogenous antitumor agents with sizes smaller than the pore size could passively diffuse into the cytoplasm in–vitro via pores created by USMB. However, these observations were made in a simple in–vitro setting with monolayers of cells cultured in a fluid environment. It is not clear whether USMB also causes pore formation in–vivo, where tumor parenchymal cells are located in a densely packed solid tissue environment.
In addition to passive diffusion through nonspecific pores on tumor cell membranes, USMB has also been shown to assist in active drug transport mechanisms into the cytoplasm, such as endocytosis, especially for larger molecules (>500 kDa). Meijering et al. (2009) and Juffermans et al. (2014) demonstrated that cellular uptake of larger molecules relied on endocytosis alone; whereas cellular uptake of smaller molecules involved both pore formation and endocytosis. Chuang et al. (2014) used microscopy to show the endocytotic process (24 h) by which albumin-shelled microbubbles loaded with paclitaxel (1.91 μm) enter breast cancer cells in presence of acoustic exposure, resulting in increased transport of albumin microbubbles into tumor cells. The exact mechanism behind USMB-induced endocytosis has not been completely elucidated. It has been speculated that ultrasound exposure and microbubble cavitation trigger changes in the membrane ion channels and the cytoskeletal arrangement, leading to an increase in intracellular Ca2+ (Parvizi et al. 2002) and polymerization of microtubules (Hauser et al. 2009). The changes may lead to enhanced endocytotic activity, thereby causing an increase in extracellular drug uptake in insonated cells. A detailed description on the microbubble-membrane interaction can be found in previous chapters of this book.
Indeed, the interaction between microbubbles and cells occurs within close proximity to the cells (Tzu-Yin et al. 2014). In–vivo, since microbubbles are spatially limited within the vessels, USMB induced in the vessel may only affect very few parenchymal cells near the vasculature (Ward et al. 2000). Therefore, successful drug delivery into distant parenchymal cells may require combination of USMB and other slow release therapeutic carrier systems. While USMB allows for passage of the therapeutic carriers across the vessels, the extravasated therapeutic carriers diffuse into the tumor parenchyma and slowly release the drug payload into tumor cells (Cochran et al. 2011a).
15.2.2 Destruction of Tumor Vascular
An alternative approach to cancer therapy is to destroy tumor vasculature by USMB. Exposure of tumor vessels to oscillating and imploding microbubbles can not only increase vascular endothelial cell membrane permeability, thereby enhancing uptake of anti-tumoral or anti-angiogenic in the vessels, but can also directly and mechanically destroy tumor vasculature. Both phenomena can cause blood vessel shut down with decreased supply of nutrients to tumor tissue (Molema et al. 1998).
15.2.2.1 Enhanced Cellular Uptake of Drugs in Vascular Endothelial Cells
The ultrasound-microbubble-cell interaction in the lumen of tumor vessels can selectively stimulate uptake of cytotoxic or anti-angiogenic drugs in vascular endothelial cells, leading to cellular apoptosis and subsequent disruption of the tumor vasculature (Fig. 15.2a).
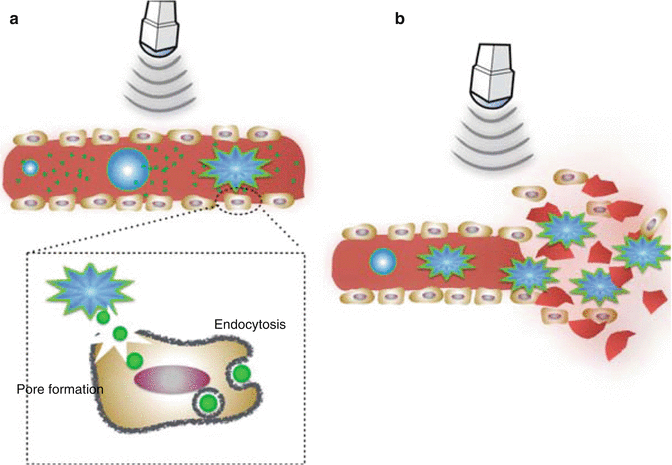
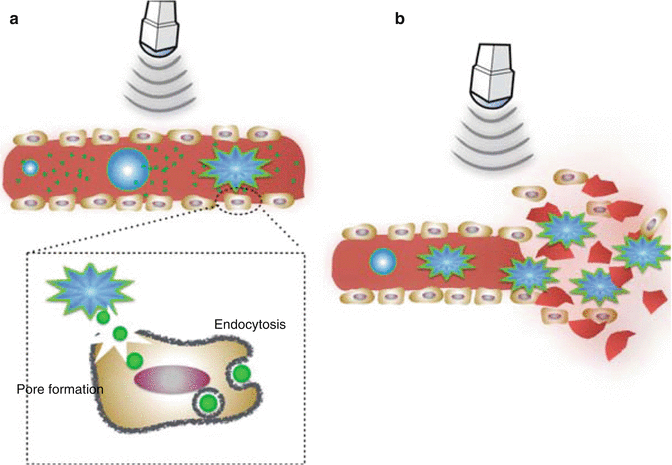
Fig. 15.2
Schematic drawing of destruction of tumor vasculature in USMB treatment. (a) Ultrasound-microbubble-cell interaction in the lumen of tumor vessels can stimulate uptake of cytotoxic or anti-angiogenic drugs in vascular endothelial cells by either creating pores on the cellular membrane and/or stimulating active transport, such as endocytosis. (b) USMB alone can directly produce an anti-angiogenic effect by mechanically disrupting tumor vasculature
In–vitro, this enhanced endothelial cellular uptake has been demonstrated using a dye, as well as fluorescently labeled molecules with difference sizes, such as propidium iodide (0.8 nm) (van Wamel et al. 2006), DiI (1 nm) (Patil et al. 2011), dextran (4.4 kDa) (Meijering et al. 2009), 5-carboxytetramethylrhodamine labeled small interfering RNA (siRNA about 15 kDa) (Juffermans et al. 2014), fluorescein isothiocyanate (FITC)-labeled dextran (500 kDa) (Taniyama et al. 2002) and Cy3-labeled plasmid DNA (about 3,500 kDa) (van Wamel et al. 2004). In–vivo, Fujii et al. (2013) demonstrated enhanced uptake of plasmid in endothelial cells in a heterotopic mammary adenocarcinoma model. In this study, vascular endothelial growth factor receptor-2 (VEGFR2) short hairpin (sh)RNA plasmid delivered by USMB (1.3 MHz, 0.9 W power, 10 s pulsing intervals for 20 min; cationic lipid-shelled microbubbles) resulted in increased knockdown of VEGFR2, as examined by PCR, immunostaining and western blotting. In–vivo contrast-enhanced ultrasound imaging further confirmed decreased tumor microvascular blood volume and blood flow in tumors treated with plasmid and USMB compared to tumors treated with plasmid alone.
15.2.2.2 Mechanical Destruction of the Tumor Vasculature
In addition to delivering anti-angiogenic therapeutics, USMB alone without adding therapeutic agents has been shown to have a direct anti-angiogenic effect in tumors (Fig. 15.2b). Wood et al. (2008) observed an acute shutdown of blood flow (as measured by Power Doppler) along with increased necrosis and apoptosis by histology following the administration of Definity® microbubbles and low intensity ultrasound (1 MHz at 2.2 W.cm−2 or 3 MHz at 2.4 W.cm−2; treatment for 3 min) in a murine melanoma model. Similarly, an acute decrease of blood flow by USMB alone (1 MHz, 0.1 ms pulse length, 1.6 MPa, Definity® microbubbles) was demonstrated in an in–vivo breast cancer model by Todorova et al. (2013). In this study, vessels in the tumor center were more preferentially disrupted versus those in the tumor periphery.
15.3 Treatment Protocols in Preclinical Experiments
USMB treatments require sufficient accumulation of microbubbles and drugs and appropriate ultrasound waves at the target tissues (Panje et al. 2012). The microbubbles and drugs can be delivered through different routes (intravenous (i.v.), intratumoral (i.t.) or intraperitoneal (i.p.)). The drugs can be mixed in the microbubble solution prior to administration or loaded on the microbubbles. Once the microbubbles and drugs reach the target tissues, appropriate ultrasound waves need to be delivered in a timely manner to produce optimal release of drugs (Willmann et al. 2008, Fig. 15.3).
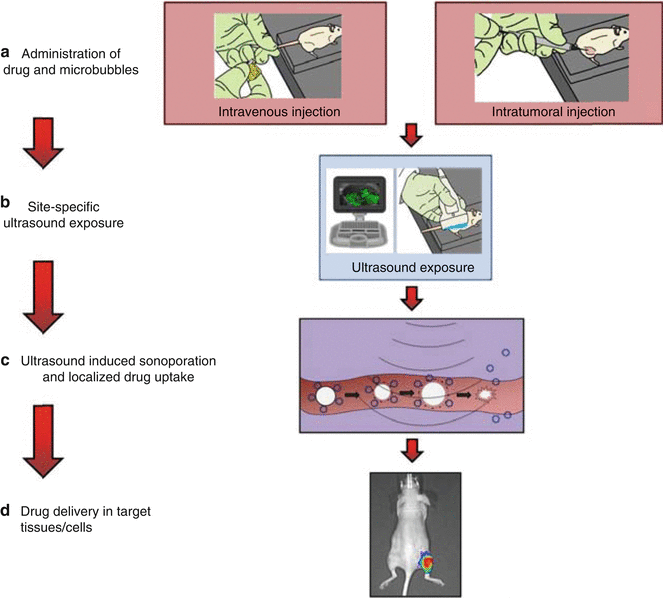
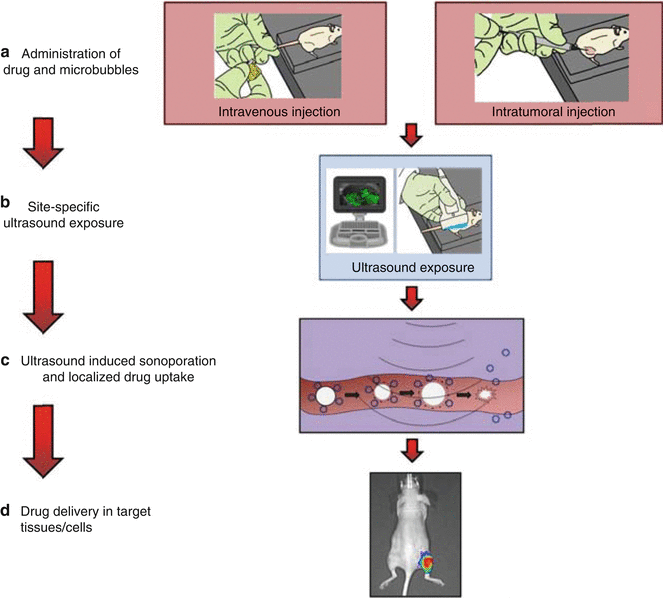
Fig. 15.3
Typical treatment protocol of USMB-mediated drug delivery in preclinical in–vivo experiments. (a) Microbubbles and therapeutic agents are administered either systemically via intravenous injection or locally through, for example, intratumoral injection. (b, c) Site-specific ultrasound exposure with the presence of microbubbles triggers sonoporation, facilitating the uptake of therapeutic agents. (d) Drug delivery outcomes could be quantified and monitored noninvasively using, for example, bioluminescence imaging shown here or other imaging modalities. Here, focal bioluminescent signal on the right hind limb of a mouse shows successful delivery of a reporter gene to the right hind limb tumor, while no imaging signal is observed elsewhere, demonstrating site-specific delivery limited to the region of insonation (This figure is adapted with permission from Panje et al. (2013))
In general, USMB treatment protocols involve systemic or local administration of microbubbles, along with a combination of therapeutic agents, followed by imaging-guided application of extracorporeal acoustic energy to actuate sonoporation at the desired delivery site. Delivery outcomes can be influenced by several factors, including but not limited to (1) whether drugs are mixed with or loaded on microbubbles, (2) routes of microbubble and drug administration, (3) ultrasound parameters, and (4) the temporal sequence of treatment. Several studies have investigated the influence of these factors on drug delivery efficiency in order to optimize treatment outcomes in cancer and to ultimately prepare this technique for clinical translation.
15.3.1 Mixing Drugs with Microbubbles Versus Loading a Drug onto Microbubbles
15.3.1.1 Mixture of Drug and Microbubbles
One approach to USMB-mediated drug delivery is co-injecting a mixture of microbubbles and therapeutic agents. The advantage of this approach is the accessibility of already commercially available clinical grade microbubbles, such as Optison®, Definity® and Lumason®, all of which are FDA-approved for clinical contrast-enhanced ultrasound imaging. Sorace et al. (2012) intravenously injected Taxol, a chemotherapeutic for breast cancer, along with Definity® in breast cancer bearing xenografts in mice followed by ultrasound insonation (1.0 MHz, 5 s pulse repetition period, mechanical index 0.5, 20 % duty cycle for 5 min). Over a 3-week treatment period, these tumors showed almost a 40 % increased inhibition and a higher degree of necrosis compared to the tumors treated with drugs alone. Wang et al. 2013a intravenously injected SonoVue® along with a suicide gene, herpes simplex virus-thymidine kinase gene (HSV-TK), for USMB treatment (mechanical index 1.2 for 10 min). They observed that USMB resulted in a 47-fold enhanced TK mRNA expression and a more than 2-fold apoptosis rate in an ovarian cancer model in mice. More examples (Sorace et al. 2012; Duvshani-Eshet et al. 2007; Matsuo et al. 2011; Carlisle et al. 2013; Wang et al. 2013a, b; Nie et al. 2008; Liao et al. 2012; Kotopoulis et al. 2014; Zhao et al. 2012; Suzuki et al. 2010; Yamaguchi et al. 2011; Heath et al. 2012; Iwanaga et al. 2007) are summarized in Table 15.1.
Table 15.1
Examples of animals studies using ultrasound and microbubbles to deliver drugs/genes for cancer therapy
Cancer types | References | Animal models | Drugs | MBs | Techniques | Routes | Treatment outcomes |
---|---|---|---|---|---|---|---|
HCC | Cochran et al. (2011b) | sc 3924a rats | DOX | Drug-loaded polymer MBs | 1 MHz, MI 0.4–0.45, 20 min | i.v., MBs, single | Drug concentration in liver tumors was increased by 7-fold compared to administration of free DOX, while drug concentrations in plasma and myocardium were reduced to 1/5 and 1/2, respectively. |
Nie et al. (2008) | sc Hepa1-6 mice | HSV-TK pDNA GCV | SonoVue® | 1 MHz, 50 % DC, 2 W/cm2, 5 min | i.v., MBs, pDNA i.p. GCV daily for 10 days | Tumor volume was approximately 1/2 of control group treated with TK and MBs at day 28. MST was significantly longer than control (survival ratio at day 100 was 50 % vs. 0 %) | |
Zhou et al. (2010) | sc H22 mice | HSV-TK pDNA GCV | pDNA-loaded lipids MBs | 1 MHz, 2 W/cm2, 5 min | i.v., MBs, pDNA i.p. GCV daily for 14 days | Tumor growth was further inhibited by 37 % compared to HSV-TK pDNA alone. Longer MST and better life quality in an 80-day continuous observation period. | |
Yu et al. (2013) | sc HepG2 mice | HSV-TK pDNA Timp3 pDNA | Liposomes MBs | 1.3 MHz, MI 1.3, 1 s interval time for 5 min | i.v., MBs, pDNA i.p. GCV daily for 4 days | Tumor growth was further inhibited by 30 % in treatment with both pDNA and USMB compared to treatments with single gene under the same USMB settings. | |
PC | Kotopoulis et al. (2014) | Orthotopic MIA PaCa-2 mice | Gemcitabine | SonoVue® | 1 MHz, MI 0.2, 40 % DC, 10 min | i.v., MBs i.p. Gemcitabine weekly for 8 wks | The primacy tumor presented only 1/3 size of those treated with drug alone. A slower onset of metastatic development was observed. |
HCC LC Glioma | Liao et al. (2012) | sc/orthotopic BNL mice Orthotopic LL/2 mice Orthotopic RT-2 mice | Endostatin Calreticulin pDNA polytreated with DOX, GM-CSF or IL-12 | SonoVue® | 1 MHz, 0.4 W/cm2, 20 % DC, 200 Hz PRF | i.m., MBs, pDNA, intermittent (weekly for 4 wks) or consecutive (daily for the initial 4 days) i.p. DOX twice i.t. GM-CSF & IL-12 single | Tumors significantly regressed in all groups. Intermittent regimen was much more effective than consecutive regimen. Administration of multiple therapeutic agents resulted in better treatment outcome than administration of single agent. |
BC | Sorace et al. (2012) | sc 2LMP mice | PTX | Definity® | 1 MHz, 5 s PRP, 20 % DC, 5 min, MI 0.1, 0.5, 1 or 2 | i.v., MBs, PTX twice a wk for 3 wks | All 4 pressure levels resulted in decreased tumor growth. Among them, MI of 0.5 resulted in the highest percentage of necrosis. |
Yan et al. (2013) | sc 4 T1 mice | PTX | Drug-loaded liposomes MBs | 2.25 MHz, 1 % DC 1 Hz PRF, 10 ms BL, 1.9 MPa, 10 min | i.v., MBs, 3 more times at 3-day intervals | Drug accumulation was 3.54- or 4.31- fold higher in tumors compared to the groups without MBs or ultrasound, while the accumulation was lower in liver & kidney. | |
Zhao et al. (2012) | sc MDA-MB-435 mice | Liposomal-DOX | Lipids MBs | 1 MHz, 0.3 W/cm2 50 % DC, 10 s | i.v., MBs, drug every 2 days | Tumor growth was inhibited using either of the three treatment schedules (USMB applied 2 h before, 2 h after, or simultaneously with drug administration), although the effect in the group treated with USMB applied 2 h after drug administration was inferior to the others. | |
BC | Carlisle et al. (2013) | sc ZR75-1 mice | Oncolytic adenovirus (polymer) | SonoVue® | 0.5 MHz, 50,000 cycles/pulse length, 0.5 Hz PRF, 1.2 MPa, 4 min | i.v., MBs & polymer, single | Circulation half-life of oncolytic adenovirus was improved by more than 50-fold. Tumor infection was enhanced by more than 30-fold, resulting in improved tumor growth retardation and prolonged MST. |
OC | Suzuki et al. (2010) | sc OV-HM mice | IL-12 pDNA | Liposome MBs | 1 MHz, 0.7 W/cm2, 1 min | i.t., MBs, pDNA single & 12-day repetitive treatments | Tumor growth was suppressed in both treatments. Complete tumor regression in 80 % of mice receiving repetitive treatments. |
Pu et al. (2014) | Orthotopic A2780/DDP mice | PTX | LHRHa-targeted drug-loaded MBs | 0.3 MHz, 1 W/cm2 50 % DC, 3 min | i.p., MBs every 3 days for 15 days | Tumor cell apoptosis increased by 38 %, angiogenesis reduced by 39 % in comparison to the non-targeted PTX-loaded MBs + US group. | |
Prostatic Cancer | Goertz et al. (2012) | sc PC3 mice | Docetaxel | Polymer MBs | 1 MHz, 50 ms burst, 1.65 MPa, 3 min | i.v., MBs & drug, single & 4-week repetitive treatment | Compared to drug alone, single treatment of USMB and drug induced a four-fold increase in necrosis, and repetitive treatment delayed tumor growth with prolonged doubling time from 0.1 to 6.9 weeks. |
Duvshani-Eshet et al. (2007) | sc PC2 mice | Hemopexin-like domain fragment pDNA | Optison® | 1 MHz, 2 W/cm2, 30 % DC 20 min | i.t., MBs, pDNA single & 4-wk repetitive treatments | Tumor growth was inhibited by 50 % after single treatment and by 80 % after repetitive treatments. | |
Greco et al. (2010) | sc DU-145 DU-Bcl-xL mice | Cancer terminator virus | Virus-loaded targeson | MI 0.7 m 1.8 MPa, 10 min | i.v., MBs weekly for 4 weeks | Primary and metastatic tumors and therapy-resistant tumors were completely eradicated. No tumor regrowth occurred 3 months after cessation of the therapy. | |
Haag et al. (2006) | sc LNCaPbl mice | Androgen receptor AO | AO-loaded lipid MBs | 1.5, 2.5 or 7 MHz, MI 1.9, 9 min | i.t., & i.v., MBs single | Stronger gene uptake in tumor tissue was detected after gene-loaded MB injection and ultrasound compared to MB complex alone (16 ~ 49 % vs. 2 ~ 18 %). | |
Melanoma | Matsuo et al. (2011) | sc C32 mice | Melphalan | Sonazoid® | 1.011 MHz, 0.064 W/cm2, 0.5 Hz burst rate, 50 % DC, 2 min | i.t., MBs, drug. Every 2 days for 2 weeks | Tumor growth ratio was significantly reduced by nearly 2.5 fold compared to drug alone. |
Yamaguchi et al. (2011) | sc C32 mice | IFN-β pDNA | Sonazoid® | 1.011 MHz, 0.22 W/cm2, 50 % DC, 3 min | i.t., MBs, pDNA, weekly for 4 weeks | Tumor growth ratio was significantly reduced by 2-fold and nearly 1.5-fold compared to blank control and gene alone, respectively. | |
SCC | Heath et al. (2012) | sc SCC-5 mice | Cisplatin Cetuximab | Definity® | 1 MHz, MI 0.5, 5 s PRP, 20 % DC, 5 min | i.v., MBs & drugs, twice weekly for 4 weeks | Tumors treated with USMB and drug exhibited a 21 ~ 26 % decrease in tumor size compared with drug alone. |
Iwanaga et al. (2007) | sc Ca9-22 mice | Bleomycin Cdt-B pDNA | Optison® | 1 MHz, 2 W/cm2, 50 % DC | i.t., MBs, pDNA. Every 2 days in the first and third wks | Tumor was completely suppressed at the end of experimental period (56 days) in the group of cdtB pDNA and USMB, and nearly disappeared in bleomycin and USMB. | |
Carson et al. (2012) | sc SCC-VII mice | EGFR-siRNA | Gene-loaded lipids MBs | 1.3 MHz, MI 1.6, 30 min | i.v., MBs, 3 times | EGFR knockdown was distributed widely (80 %) throughout treated tumors. Tumor doubling time was prolonged from 2.7 to 8.5 days compared to gene alone. | |
Glioma | Liu et al. (2010) | Orthotopic C6 rats | Carmustine | SonoVue® | 0.4 MHz, 0.62 MPa, 10 ms burst length, 1 Hz PRF, 30 s | i.v., MBs & drug, single | Drug delivery was enhanced by two-fold. Tumor growth was inhibited. MST was prolonged from 33 to 53 days compared to drug alone. |
Treat et al. (2012) | Orthotopic 9 L rats | Liposomal-DOX | Definity® | 1.7 MHz, 1.2 MPa, 10 ms burst length, 1 Hz PRF for 1–2 min repeated every 5 min | i.t., MBs, drug, single | Tumor doubling time was prolonged from 2.7 days to 3.7 days compared to drug alone. MST was improved. | |
Ting et al. (2012) | Orthotopic C6 rats | Carmustine | Drug-loaded MBs | 0.7 MPa, 10 ms burst length, 5 % DC, 5 Hz PRF, 1 min | i.v., MBs, twice | Circulation drug half-life was prolonged by four-fold. Drug accumulation in the liver was reduced fivefold compared to drug alone. Tumor growth ratio was significantly reduced from 117.4 to 39.6 % and MST was improved from 29.5 to 32.5 days compared to drug alone. |
15.3.1.2 Drug-Loaded Microbubbles
The drawbacks of co-injecting drugs freely along with microbubbles are (1) faster degradation of certain drugs (nucleic acid (Zhou et al. 2010; Greco et al. 2010; Haag et al. 2006) and RNA (Carson et al. 2012)), and (2) potentially increased toxicity to other organs (other than liver and spleen in which microbubbles are usually cleared). To address these issues, microbubbles have been exploited as drug delivery vehicles as they are amenable for surface modification. Several strategies have been proposed for conjugating therapeutic agents onto or into microbubble carriers, which are further detailed in Chap. 11. For example, drugs can be embedded within the microbubble shell, dissolved in an oily layer between the gas core and the shell, or linked to the surface of the microbubbles. However, the drug loading capacity using these approaches is generally low. To improve this, alternative techniques coupling drug loaded liposomes or nanoparticles onto the microbubble shell have been reported (Wang et al. 2012; Sirsi SR and Borden MA (2014) State-of-the-art materials for ultrasound-triggered drug delivery. Advanced drug delivery reviews.
15.3.2 Routes of Microbubbles and Antitumor Agent Administration
Several drug administration routes have been explored including i.v., i.t., and i.p. injections. Direct i.t. and i.p. injection allow delivery of high local concentrations but are invasive.
15.3.2.1 Intravenous Injection
In most studies on USMB-mediated drug delivery for cancer therapy in preclinical animal models, microbubbles and drugs are administered intravenously. The potential drawbacks of this approach are the potential systemic toxicity and, in the case of gene delivery, the rapid degradation of the agent in the circulation. Both disadvantages can be overcome by either attaching drugs directly onto microbubbles or by loading them into nanoparticles.
Another potential drawback of systemic administration is that the delivery efficiency may be limited in hypovascularized tumors, such as pancreatic cancer (Fukumura and Jain 2007), because this route relies on sufficient tumoral vascularity for circulating microbubbles and drugs to float into target lesions.
15.3.2.2 Intratumoral Injection
Several early proof-of-concept animal studies have used this approach and demonstrated improved drug delivery after ultrasound exposure (Duvshani-Eshet et al. 2007; Iwanaga et al. 2007; Haag et al. 2006). The main advantage of i.t. injection is the ability to deliver high concentrations of drugs directly to the site of desired treatment while minimizing systemic toxicity (Duvshani-Eshet et al. 2007). However, this method of administration is invasive and can be challenging if the target lesion is located in an area that is difficult to access.
15.3.2.3 Intraperitoneal Injection
This approach may be useful for primary peritoneal cancers or cancers with i.p. spread as local drug concentration can be increased at the tumor sites (Pu et al. 2014; Kotopoulis et al. 2014). It has been shown that i.p. injection of drugs can result in 20- to 1,000-fold higher peritoneal drug concentrations compared to plasma concentrations (Zimm et al. 1987; Markman et al. 1992). Micron-sized microbubbles, injected intraperitoneally, can stably persist in the peritoneal cavity without rapid clearance through the lymphatic drainage (Pu et al. 2014; Kohane et al. 2006; Tsai et al. 2007). Recently, in a mouse model of metastatic peritoneal lesions of ovarian cancer, Pu et al. (2014) injected luteinizing hormone-releasing hormone (LHRH) receptor-targeted paclitaxel-loaded microbubbles into the mouse peritoneal cavity and exposed the abdomen to ultrasound for sonporation. These microbubbles specifically bind to tumor cells expressing LHRH, and upon exposure to ultrasound, encapsulated drugs can be locally released at the tumor sites. Compared to treatment with paclitaxel alone, this approach resulted in an approximately two-fold higher apoptosis rate, an extended median survival time of treated mice from 37 to 47 days, and an approximately 55 % reduced tumor angiogenesis. In patients, however, peritoneal spread of cancer is usually diffuse and further studies are needed to assess clinical practicability of this approach to efficiently treat a diffuse disease process, such as peritoneal carcinomatosis using USMB.
15.3.3 Ultrasound Parameters
Most studies have shown successful USMB-guided drug delivery using already available clinical ultrasound imaging systems; however, the reported delivery efficiency is inconsistent (Newman and Bettinger 2007), likely due to there being so far no standardization of the acoustic parameters. To date, standardized ultrasound parameters for drug delivery have not been determined on any of the current clinical ultrasound systems, possibly because the systems have limited tunable acoustic parameters. This makes it difficult to perform a systematic and parametric study for optimal drug delivery on these systems. Optimizing ultrasound parameters tailored for the purpose of drug delivery has the potential to improve treatment outcomes (Yu et al. 2013). To determine an optimal setting for effective delivery, some studies compared drug delivery outcomes using custom-built ultrasound systems with more flexibility in the acoustic parameters. A wider range of ultrasound parameters was tested in drug delivery into cells in–vitro (Sonoda et al. 2007; Ghoshal et al. 2012) and in–vivo (Sorace et al. 2012; Wang et al. 2013a, Haag et al. 2006). So far, standard ultrasound parameters for USMB drug delivery have not been established. In–vivo ultrasound settings suggested by current literature are as follows:
Ultrasound frequency: 0.4 ~ 3 MHz. Lower frequencies are more preferable in general because the pressure threshold to initiate cavitation is reduced in the low frequency range (Apfel and Holland 1991).Stay updated, free articles. Join our Telegram channel
Full access? Get Clinical Tree
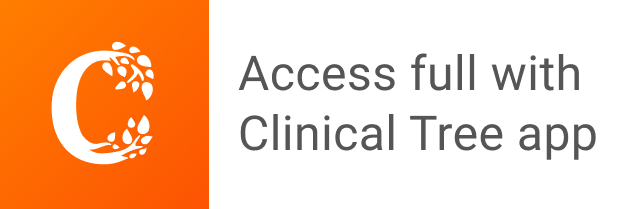