Fig. 19.1
Microbubble tunneling though a fibrin clot (fluid-clot boundary at left) caused by acoustic radiation force (1 MHz, 0.4 MPa). The blurred, dark line is a motion artifact from deflection of the clot boundary due to radiation force (Acconcia et al. 2013)
19.2.3 Secondary Mechanical Effects (Acoustic Cavitation)
19.2.3.1 Classification of Cavitation
Acoustic cavitation has been a topic of study since the early twentieth century (Rayleigh 1917) and has been extensively reviewed elsewhere (Flynn 1964; Apfel 1981; Leighton 1994; Lauterborn and Kurz 2010). Acoustic cavitation refers to both the formation and oscillation of bubbles due to an acoustic pressure. Cavitation activity can generally be categorized as inertial or stable.
Inertial cavitation encompasses bubble motion dominated by the inertia of the surrounding fluid. Large expansions of the bubbles occur due to relatively large tension generated in the liquid by the acoustic excitation (Holland and Apfel 1989). During the final stages of bubble collapse, the converging liquid compresses and heats the contents of the bubble, creating a high energy density (Young 2005). The sudden halt of the converging liquid generates shock waves (Holzfuss et al. 1998), light emissions (Gaitan et al. 1992), and free radicals (Riesz and Kondo 1992). If the collapse occurs along a surface, a liquid jet with speeds in excess of 1 km/s may form (Brujan et al. 2001). These jets are associated with mechanical damage or deformation of clots (Weiss et al. 2013) (Fig. 19.2a).
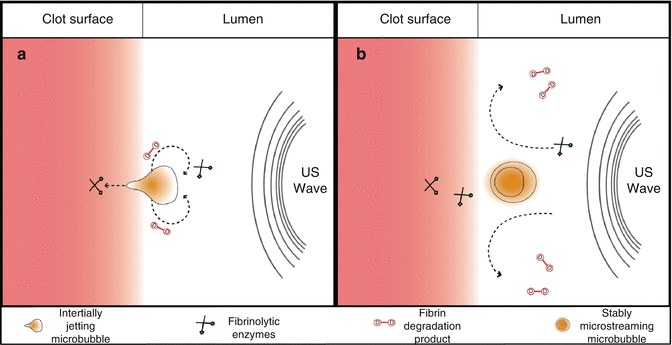
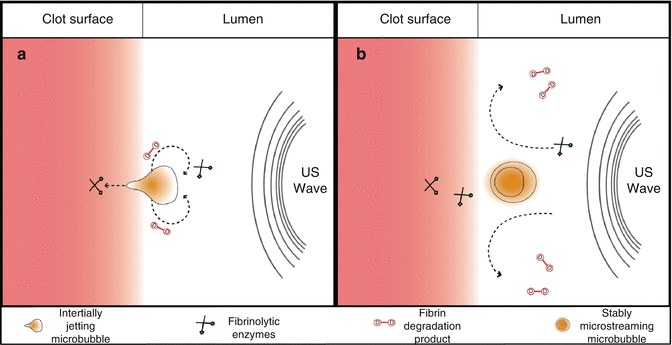
Fig. 19.2
Interaction of cavitating microbubbles with thrombi. (a) Illustration of inertial cavitation. The asymmetric boundary condition causes a liquid jet to form during the final stages of collapse during inertial cavitation (Brujan et al. 2001). The jet will impinge on the thrombus, resulting in direct mechanical damage and erosion of clot surface. (b) Illustration of stable cavitation. Sustained bubble motion promotes strong fluid mixing through microstreaming (Elder 1959). Local pressure gradients around the microbubble cause enhanced mixing of the fibrinolytic enzymes, and removal of fibrin degradation products from the clot (Datta et al. 2008) (Figure Adapted from Sutton et al. (2013a))
Chen et al. (2014) used high-speed imaging to observe the formation of a defect in a thrombus surface at the location of a microbubble jet after ultrasound exposure (Fig. 19.3). Inertial cavitation can be detected acoustically as broadband emissions (Everbach and Francis 2000; Datta et al. 2008). Chuang et al. (2010) found a positive correlation between the dose of broadband acoustic emissions and the thrombolytic efficacy in-vitro. Similarly, Leeman et al. (2012) observed significant fibrinolysis in-vitro only when insonation of microbubbles produced broadband acoustic emissions. Broadband acoustic emissions have also been detected in-vivo during thrombolysis in porcine (Shi et al. 2011; Maxwell et al. 2011) and rabbit (Wright et al. 2012a) models. Xie et al. (2009) found significant thrombolytic enhancement in a canine model only when microbubbles nucleated inertial cavitation. However, Wu et al. (2014) found that thrombolytic efficacy did not correlate with the amplitude of in-vitro broadband acoustic emissions. They concluded instead that strong inertial cavitation shielded the acoustic energy from the thrombus. Although strong inertial activity appears to enhance thrombolysis in some cases (Chen et al. 2009; Maxwell et al. 2009), sustained bubble activity is difficult (Prokop et al. 2007), potentially due to the destruction of cavitation nuclei (Datta et al. 2006).
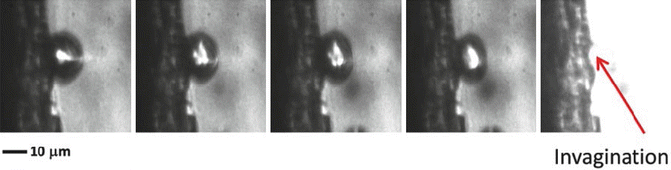
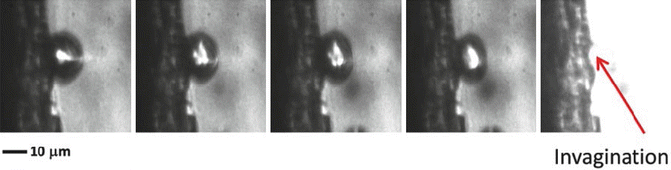
Fig. 19.3
Interaction of inertially cavitating microbubble with thrombus (1 MHz, 1.5 MPa) recorded at 5 Mfps (200 ns interframe time). As the microbubble disappears at the completion of the inertial collapse, there is a residual “pit” at the thrombus site (Chen et al. 2014)
Stable cavitation is a sustained bubble motion (Datta et al. 2006) where the converging liquid inertia is offset by the restoring force of the gaseous contents of the bubble (Flynn 1964). The acoustic pressure amplitude required to initiate stable oscillations is generally lower than that required for inertial cavitation (Bader and Holland 2012). The highly nonlinear nature of these oscillations generates microstreaming of the surrounding fluid (Elder 1959), promotes strong fluid mixing (Collis et al. 2010) and attracts particulates via secondary radiation force (Nyborg and Miller 1982). Such strong fluid mixing hastens enzymatic fibrinolysis, enhancing the penetration of both rt-PA and plasminogen into clots (Datta et al. 2008; Sutton et al. 2013a) (Fig. 19.2b).
In contrast to the broadband emissions from inertial cavitation, stable cavitation generates harmonic, ultraharmonic, and subharmonic emission lines in the acoustic spectra. Depending on the relative size of the bubble compared to the resonant size (Bader and Holland 2012), harmonic (integer multiples of the fundamental) (Choi and Coussios 2012), subharmonic (rational fractions of the fundamental less than one) (Prokop et al. 2007), or ultraharmonic (rational fractions of the fundamental greater than one) (Datta et al. 2006) emissions can be used to detect stable cavitation acoustically. Datta et al. (2008) found a correlation between thrombolytic enhancement and ultraharmonic emissions in-vitro. Subsequent studies from the same group (Hitchcock et al. 2011) found increased thrombolytic efficacy using an intermittent insonation scheme that optimized ultraharmonic emissions from stable cavitation.
Bader et al. (2015)) used an in-vitro model to monitor the instantaneous reduction in clot width and ultraharmonic emissions from stable cavitation nucleated from Definity® microbubbles. The energy of ultraharmonic emissions was found to correlate significantly with the instantaneous reduction in clot width (i.e., thrombolytic efficacy). Prokop et al. (2007) suggested a correlation between increased thrombolytic efficacy in-vitro and the presence of subharmonic emissions from stable cavitation. The correlation between subharmonic or ultraharmonic emissions established by Prokop et al. (2007), Datta et al. (2008), and Bader et al. (2015)) illustrate the importance of stable cavitation in assisting enzymatic fibrinolysis.
To gauge both types of cavitation activity incited, Apfel (1981) recommends the use of three golden rules: Know thy sound field, Know thy liquid, and Know when something happens. These rules can help optimize sonothrombolysis treatments employing acoustic cavitation.
19.2.3.2 Know Thy Sound Field
Consistent insonification is necessary for safe and efficient sonothrombolysis. Several ultrasound exposure approaches have been proposed, as summarized in Fig. 19.4.
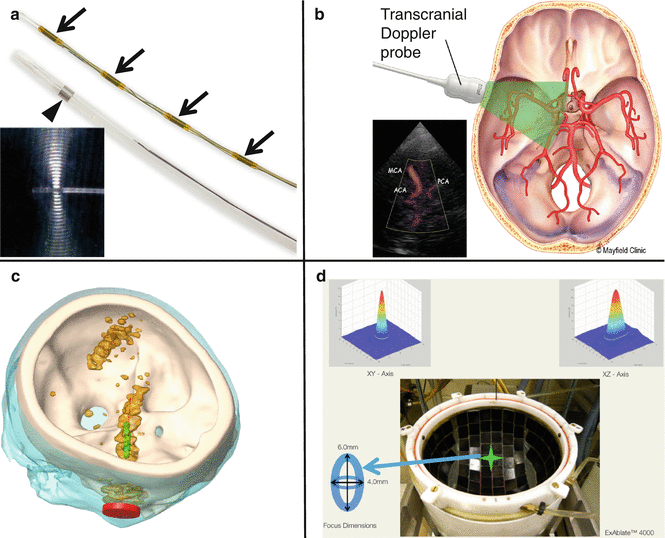
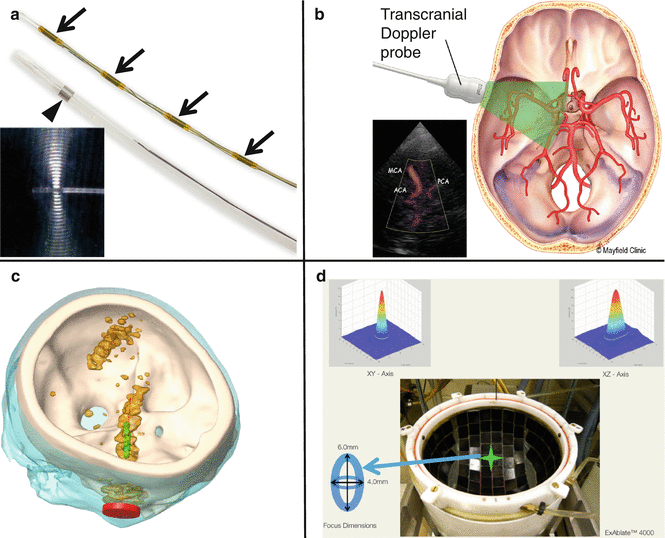
Fig. 19.4
Ultrasound insonation schemes for sonothrombolysis. (a) Ultrasound-assisted thrombolysis catheter composed of a 5.2-Fr multi-sidehole drug infuser (arrowhead). Ultrasound elements along central core (small arrows) shown separately. During treatment, the central core is placed inside the infusion catheter (Engelberger and Kucher 2014). The omnidirectional ultrasound field promotes cavitation and acoustic streaming, driving thrombolytics into the clot. Images provided by EKOS Corporation (Bothell, WA, USA). (b) Transcranial Doppler. Schematic representation of the penetration of 2-MHz TCCS through the skull. Images reprinted with permission from Mayfield Clinic (Cincinnati, OH, USA). (c) Unfocused, Sub-megahertz Ultrasound. Simulated acoustic fields generated by an unfocused, transcranial sub-megahertz (120 kHz) ultrasound system in a human skull (white). The M1 segment of the MCA is shown in green and the transducer in red. The orange contour indicates the regions where the acoustic pressure is larger than half the maximum pressure in the M1 region of interest (Bouchoux et al. 2014). (d) MR-Guided Focused Ultrasound. Top view of ExAblateTM 4000 HIFU hemispherical 1000-element system for MR-guided transcranial focused ultrasound exposure. A sharp 4 mm × 6 mm focus is created along the lateral and elevational orientations, respectively (Hölscher et al. 2013)
19.2.3.2.1 Ultrasound Catheter
Ultrasound-emitting catheters have been applied to a wide variety of pathologies (Doomernik et al. 2011). The catheter is inserted into the thrombus allowing combined exposure to ultrasound and local drug infusion. A relatively uniform ultrasound field covering large clots can be obtained using a series of miniaturized unfocused transducers aligned in the lumen of the catheter (Soltani et al. 2007). The acoustic pressure decreases rapidly with distance from the catheter because the transducers are small and unfocused. Nevertheless, effective ultrasound-catheter sonothrombolysis was achieved in large thrombi caused by intracerebral hemorrhage (Newell et al. 2011).
19.2.3.2.2 Transcranial Doppler
Extracorporeal insonation of the thrombus, combined with an intravenous injection of a thrombolytic drug and/or microbubbles, is an appealing minimally invasive approach for the treatment of ischemic stroke. The cranial bone attenuates, reflects and distorts the ultrasound waves (Fry et al. 1970). Hence, bone constitutes a major obstacle for transcranial sonothrombolysis. The temporal bone acoustic window is routinely used for transcranial Doppler (TCD) examination of cerebral blood flow (Aaslid et al. 1982). TCD, or transcranial color-coded sonography (TCCS), exposure through the temporal bone accelerates rt-PA thrombolysis (Cintas et al. 2002; Alexandrov et al. 2004; Eggers et al. 2008). TCD and TCCS sonothrombolysis use similar frequencies (around 2 MHz) and similar pulsed-ultrasound waveforms, and comparable results were obtained in clinical trials (Saqqur et al. 2013).
TCD is operator dependent, and the lack of trained operators is an obstacle for widespread adoption (Barlinn et al. 2012). To overcome this limitation, a sonothrombolysis device has been designed in order to be simpler to put into place and is less operator-dependent than TCD (Barlinn et al. 2013). A frame is fixed to the patient’s head using simple landmarks. Eighteen transducers are mounted on the head frame in order to insonify the most frequent locations of thrombi during ischemic stroke through the temporal and suboccipital acoustic windows. The acoustic parameters used by this device are based on those used in FDA-approved TCD devices (2 MHz, 100 kPa derated peak rarefactional pressure). The safety and relevance of this device has been demonstrated in phase I and II clinical trials (Barreto et al. 2013).
Ultrasound waves at 2 MHz are transmitted poorly through the bone (Ammi et al. 2008), which prevents TCD examination in 18% of patients (Wijnhoud et al. 2008). Barlinn et al. (2012) estimated the in-situ acoustic pressure for 20 subjects treated with TCD in the TUCSON trial using a simple attenuation model based on CT data. The calculated in-situ acoustic pressures were higher for patients functionally independent at 3 months. Hence, some patients may receive an insufficient acoustic pressure for thrombolysis enhancement with TCD insonification.
19.2.3.2.3 Sub-megahertz Ultrasound
Efficient acoustic transmission through the skull can be achieved in the sub-megahertz frequency range. Ammi et al. (2008) measured a pressure reduction resulting from bone between 77.1 and 96.6% at 2 MHz in 5 human skull specimens. A pressure reduction between 22.5 and 45.5% was observed at 120 kHz. Therefore, sub-megahertz sonothrombolysis may allow a more consistent insonation than 2-MHz TCD. Sub-megahertz (300 kHz) insonation was used in the TRUMBI clinical trial (Daffertshofer et al. 2005). However, a significantly higher rate of symptomatic hemorrhage occurred in the ultrasound group. Baron et al. (2009) numerically simulated TRUMBI trial insonation parameters and concluded that standing waves, due to acoustic reflections in the cranial cavity, could have caused unexpected local pressure maxima. Reflection from contralateral bone and associated constructive interference are less likely for 2-MHz TCD because the wave is significantly attenuated while propagating in the brain tissue. The TRUMBI trial and subsequent studies revealed the necessity of producing a well-controlled transcranial ultrasound field for safe and efficient sonothrombolysis.
Taking into account these findings, revised sub-megahertz transcranial sonothrombolysis devices were designed. A dual-frequency array capable of performing 2.5-MHz TCCS and emitting a 500-kHz sonothrombolysis beam was developed for transcranial insonation (Azuma et al. 2010). The transcranial ultrasound field produced by this device was evaluated in-vitro and the safety of this approach was demonstrated in a healthy primate model (Shimizu et al. 2012). Bouchoux et al. (2014) simulated 120 and 500-kHz transcranial ultrasound fields from the head CT scans of 20 ischemic stroke patients using a validated acoustic propagation numerical model (Bouchoux et al. 2012). Consistent and homogeneous insonation of the M1 segment of the MCA was achieved at both 120 and 500 kHz. A positioning strategy based on external head landmarks, which did not require the knowledge of the position of the thrombus, was proposed by Bouchoux et al. and was found to perform similarly to an optimized transducer positioning technique based on CT data analysis. Local acoustic pressure maxima, due to reflection from the contralateral bone, were well controlled and similar to the amplitude in the targeted M1 segment of the MCA.
Transcranial exposure with sub-megahertz ultrasound may allow simple and consistent insonation of the thrombus in ischemic patients, without excluding subjects with an insufficient temporal bone window for TCD or TCCS. Sub-megahertz sonothrombolysis devices should be developed and evaluated carefully. The transcranial ultrasound field can be evaluated by simulation or in-vitro measurements in several skulls. Moreover, sub-megahertz sonothrombolysis devices should be evaluated in a large animal model accounting for constructive interference due to standing waves. Random frequency modulation of the wave can also be employed to suppress standing waves (Tang and Clement 2010; Furuhata and Saito 2013).
19.2.3.2.4 Focused Ultrasound
Thrombolysis with focused ultrasound is also a promising approach. A highly focused ultrasound beam allows accurate spatial targeting of the clot. High-pressure amplitudes can be applied locally with a low risk of side effects outside the focal zone. Therefore, thrombolysis based on erosion of the clot by inertial cavitation may be possible with focused ultrasound without a thrombolytic drug. Maxwell et al. (2011) treated acute thrombi in juvenile pig femoral arteries using a 1-MHz transducer with a 1.9 × 13.5 mm focus (−6 dB). Similarly, a 1.5-MHz transducer (0.9 × 7.1 mm focal zone) was used to lyse clots in rabbit femoral arteries (Wright et al. 2012a). These studies demonstrated the feasibility of high intensity focused sonothrombolysis using accurate clot targeting. However, the focal zone is usually larger than the targeted vessels. Therefore careful studies on the side effects on tissue surrounding the clot are needed. The treatment of ischemic stroke with a MR-guided transcranial focused ultrasound device (ExAblate™ 4000, InSightec Inc., Tirat Carmel, Israel) was also proposed (Hölscher et al. 2013). This device is a hemispheric 1000-element 220-kHz phased array that can produce an electronically-steered 4 × 6 mm transcranial focal spot. Nevertheless, the work reported by Hölscher et al. (2013) is in a very early stage and more studies are needed to assess the efficacy and safety of this approach. Safe and efficient intracerebral hemorrhage (ICH) clot liquefaction with a similar MR-guided transcranial ultrasound device was demonstrated in an in-vivo porcine model and in a human cadaver model (Monteith et al. 2013). Though large aperture, focused ultrasound for transcranial insonation appears promising, numerical studies by Pajek and Hynynen (2012) indicate that these devices currently appear to be limited to treatment near the center of the brain (basilar artery and the proximal end of the M1 segment of the middle cerebral artery).
19.2.3.3 Know Thy Liquid (i.e., Know Thy Cavitation Nuclei)
The type and threshold for cavitation activity incited depends highly on the nuclei that are present.
19.2.3.3.1 Endogenous Nuclei
Thresholds for cavitation activity depend on the purity of the medium (Roy et al. 1985). However, cavitation thresholds in even the purest sources of water (Herbert et al. 2006; Bader et al. 2012; Maxwell et al. 2013) are much less than theoretically predicted (Church 2002). This discrepancy is generally attributed to nanometer-sized nuclei (Bader et al. 2012; Maxwell et al. 2013) that are difficult to extract from the medium (Flynn 1964). Such nuclei are however not likely to be found in-vivo due to the body’s natural filtration system. Instead, Yount (1979) proposed nuclei composed of an elastic organic skin of amphiphilic molecules which would stabilize bubbles against dissolution. The cavitation thresholds of endogenous nuclei in tissue are summarized by Church (2015). Maxwell et al. (2013) measured the thresholds for a wide range of ex-vivo tissues with short 1-MHz pulses, including clots, using a regime of therapeutic ultrasound termed histotripsy (Xu et al. 2004). The cavitation threshold of clots was similar to that of pure water, suggesting similar nanometer-sized nuclei to that observed in water.
Cavitation from endogenous nuclei has been observed during sonothrombolysis. Maxwell et al. (2011) observed microbubble clouds as regions of hyperechogenicity using 10-MHz diagnostic ultrasound imaging while insonating thrombi in a porcine femoral vein artery at 1 MHz. Additional studies have observed acoustic emissions from inertial (Everbach and Francis 2000; Wright et al. 2012a) or stable cavitation (Datta et al. 2006) during ultrasound-enhanced thrombolysis.
19.2.3.3.2 Exogenous Nuclei
Introducing exogenous nuclei allows control of the type and location of cavitation activity. Hydrophobic particulates (Soltani 2013), perfluorocarbon droplets (Pajek et al. 2014) and focused laser pulses (Cui et al. 2013) have been used to nucleate cavitation effectively during sonothrombolysis. However, ultrasound contrast agents (UCAs), or stabilized microbubbles, are also used to nucleate cavitation. Excellent reviews of the principles of UCAs are provided by Stride and Saffari (2003) and Cosgrove (2006). The specific use of UCAs for sonothrombolysis is reviewed by de Saint et al. (2014). Briefly, most commercially available UCAs are composed of a high-molecular-weight inert gas core surrounded by a lipid shell (Faez et al. 2013). The use of a gas with low solubility in the blood stream and pegylation of the lipid shell increases the circulation lifetime (Sarkar et al. 2009). The large compressibility of the gas compared to the surrounding tissue results in a high backscatter of incident acoustic pulse. UCAs are excellent blood pooling agents for left ventricular opacification (Radhakrishnan et al. 2013), detection of focal lesions in the liver (Claudon et al. 2013) and evaluation of brain perfusion (Claudon et al. 2008). UCAs range in size from 1 to 10 μm in diameter (Bouakaz and de Jong 2007), which prevents filtration of UCAs by the lungs (Hogg 1987). In addition, UCAs of this size are resonant at diagnostic imaging frequencies (1–10 MHz). Current FDA-approved use of UCAs is restricted to left ventricular opacification, although there is a growing interest in using UCAs for therapeutic applications as well (Cosgrove and Harvey 2009; Stride et al. 2009).
To gauge the potential for generating therapeutic cavitation activity from UCAs, Bader and Holland (2012) investigated the nucleation and detection of stable cavitation numerically. The cavitation index (I CAV ) was devised to gauge the likelihood of stable cavitation activity from UCAs in terms of the peak rarefactional pressure in MPa (P r ) and center frequency in MHz (f):
Bader and Holland found there was an increased likelihood of nucleating cavitation activity by rupturing the UCA shell when
0.09 $$
” src=”https://radiologykey.com/wp-content/uploads/2017/06/A317357_1_En_19_Chapter_IEq2.gif”>. Subharmonic emissions are more likely from inertial cavitation when
19.5).
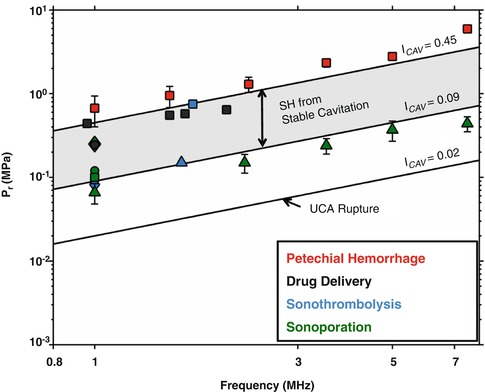

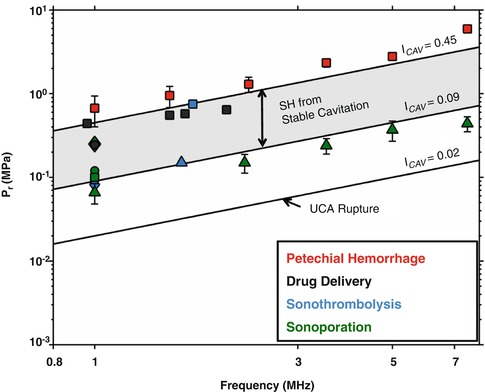
Fig. 19.5
Comparison of cavitation index to selected bioeffects (Bader and Holland 2012). The line labeled I CAV = 0.45 indicates the peak rarefractional pressure (P r ) required to initiate shell rupture of ultrasound contrast agents. The lines labeled I CAV = 0.09 and I CAV = 0.45 bordering the shaded region demarcate the parameter space over which subharmonic (SH) emissions from stable cavitation are likely. The cavitation index is well suited for predicting beneficial bioeffects associated with stable cavitation, such as drug delivery (Hitchcock et al. 2010; McDannold et al. 2008), sonoporation (Greenleaf et al. 1998; Miller and Dou 2004; Rahim et al. 2006; Juffermans et al. 2009) and sonothrombolysis (Porter et al. 2001; Prokop et al. 2007; Petit et al. 2012a). Beyond I CAV = 0.45, subharmonic emissions originate from inertial cavitation. Bioeffects associated with inertial cavitation, such as petechial hemorrhage (Miller et al. 2008), occur at a cavitation index above 0.45
19.2.3.4 Know When Something Happens
Apfel’s final golden rule refers to the detection of cavitation activity. While some in-vitro studies used high-speed imaging to monitor cavitation activity during sonothrombolysis (Caskey et al. 2009; Acconcia et al. 2013; Chen et al. 2014), such observations are not possible in-vivo. Devising methods to monitor cavitation is important to translate these treatments through the regulatory process and into the clinic (Harris 2009). The most common method of monitoring cavitation activity in sonothrombolysis studies is passive cavitation detection (Everbach and Francis 2000; Datta et al. 2006; Prokop et al. 2007), whereby cavitation acoustic emissions are passively received by a transducer. The frequency spectrum of the signal is inspected for the presence of either broadband emissions to indicate the presence of inertial cavitation, harmonic, subharmonic, or ultraharmonic emissions to indicate the presence of stable cavitation. The strength of these characteristic cavitation emissions can be correlated with thrombolytic efficacy in order to gain insight into the type of cavitation activity required to promote maximal thrombolytic efficacy. Chuang et al. (2010) found a positive correlation between the thrombolytic efficacy and the strength of broadband acoustic emissions in an in-vitro human clot model. In contrast, Datta et al. (2008) and Bader et al. (2015) found a positive correlation between thrombolytic efficacy and the strength of ultraharmonic emissions in in-vitro models.
Passive cavitation detection with single-element transducers provides limited information on the spatial distribution of cavitation activity. A new method of cavitation detection, passive cavitation imaging (PCI) (Salgaonkar et al. 2009; Haworth et al. 2012; Jensen et al. 2012), beamforms received cavitation signals from multiple-element arrays. The resulting passive cavitation images resolve the location and type of cavitation activity. Vignon et al. (2013) demonstrated the feasibility of PCI to detect and map cavitation activity in-vivo for thrombolysis studies. Shi et al. (2011) implemented the technique to monitor cavitation nucleated from Doppler exposure of Definity® in an in-vivo porcine model. Cavitation emissions mapped within the porcine skull were a mixture of both stable and inertial cavitation at the lower Doppler amplitudes (0.46 mechanical index). Only inertial cavitation was observed at the higher Doppler amplitudes (1.7 mechanical index).
19.3 Experimental Evidence for Ultrasound-Enhanced Efficacy
19.3.1 Assessment of Sonothrombolysis in the Laboratory
Since the first demonstration of ultrasound-enhanced thrombolysis by Sobbe et al. in 1974, nearly 500 studies have been published on sonothrombolysis (Scopus search engine, Accessed 9 July 2014). These studies have included “bench top” experiments, designed to elucidate and maximize the mechanisms of ultrasound that enhance thrombolysis. The conclusions of these studies depend on the type of study (i.e., in-vitro, ex-vivo, or in-vivo), modeling of biological parameters (i.e., clot manufacturing process, thrombolytic metric, etc.), and the ultrasound exposure conditions.
19.3.1.1 In-Vitro, Ex-Vivo, and In-Vivo Studies
In-vitro studies minimize the complexity associated with biological systems to assess the interaction of ultrasound with clots in a controlled setting. Clot models have been developed through strict protocols in order to produce consistent, clinically-relevant clots (Holland et al. 2008). Coagulation is initiated by incubating fresh or recalcified blood at physiologic temperature (37 °C) (Roessler et al. 2011). Coagulation can also be initiated by the addition of thrombin to plasma (Lauer et al. 1992; Suchkova et al. 1998). Fibrin clots, which are optically translucent, are also used for optical studies (Acconcia et al. 2013). The degree of clot retraction can be modified by altering the properties of the surface in contact with incubated blood (Sutton et al. 2013b), or by storing the clot at low temperatures (<4 °C) for several days post-incubation (Shaw et al. 2006). Thrombolytic metrics have included mass loss (Datta et al. 2006), dimensional reduction on an image (Cheng et al. 2005; Kim et al. 2012; Petit et al. 2012a), or the presence of fibrin degradation products (Francis et al. 1992; Kimura et al. 1994; Pfaffenberger et al. 2003; Alonso et al. 2009). Interested readers are invited to review an exhaustive list of recent in-vitro studies compiled by Petit et al. (2012b).
Ex-vivo studies incorporate excised living tissue as a first step towards understanding the interaction of vascular tissue exposed to thrombolytics and ultrasound. Excised arteries have been employed to determine the effect on the endothelium during sonothrombolysis (Rosenschein et al. 2000; Hitchcock et al. 2011). Clot models are similar to those used in-vitro, and mass loss is used as the thrombolytic metric.
In-vivo animal models allow the full biological response of a living organism to ultrasound and thrombolytics to be assessed, including the efficacy of the treatment, the ability to track and monitor treatment progress, physiologic alterations, and the potential for collateral damage. These studies are required prior to U.S. Food and Drug Administration approval for new clinical devices (Harris 2009). A variety of in-vivo thrombosis models have been developed and are summarized by Hossmann (1998), Verbeuren (2006), and Mousa (2010). Small animal models, such as rodent (Daffertshofer et al. 2004) and rabbit (Hölscher et al. 2012), are used in sonothrombolysis due to their low cost, ease of handling, and accumulation of data from previous studies. Large animal models, such as swine (Culp 2004) and primates (Shimizu et al. 2012), are needed to model the propagation of ultrasound through the skull and brain architecture. Thrombus formation can be initiated by incubation of autologous blood within a stenotic artery (Culp 2004; Damianou et al. 2014). High-intensity laser pulses can also be used to induce clot formation (Yamashita et al. 2009; Chen et al. 2013). Flow velocity (Maxwell et al. 2011), degree of thrombus size burden (Stone et al. 2007), or the presence of fibrin degradation products (Xie et al. 2005; Yamashita et al. 2009) can be used to assess thrombolytic efficacy. Potential side effects are evaluated using imaging techniques, post-mortem histology and immunohistochemistry techniques.
19.3.1.2 Ultrasound Exposure Conditions
Insonation of thrombi to initiate lysis have been employed with and without thrombolytic drugs. UCAs have also been used to nucleate cavitation activity in both cases. The degree of thrombolysis is dependent on the ultrasound exposure conditions (Holland et al. 2008; Petit et al. 2012a).
19.3.1.2.1 Ultrasound Therapy Without a Thrombolytic
In the absence of a thrombolytic drug, lysis is initiated by the mechanical interaction of ultrasound energy with the thrombus. Maxwell et al. employed 1-MHz histotripsy pulses (Xu et al. 2004) to lyse clots in-vitro completely within 5 min (Maxwell et al. 2009). This technique reduced the thrombus size in 10 of 12 cases in an in-vivo swine model (Maxwell et al. 2011). Westermark et al. (1999) determined pulsed ultrasound increased the thrombolytic efficacy compared to continuous wave ultrasound in-vitro. Similarly, Rosenschein et al. (2000) and Wright et al. (2012a) found efficient thrombolysis with pulsed ultrasound both in-vitro and in-vivo. Moderate collateral damage was observed in-vivo in the form of coagulative necrosis (Rosenschein et al. 2000), hemorrhage (Wright et al. 2012a; Burgess et al. 2012), or denudation of the endothelium (Maxwell et al. 2011). These deleterious effects could be explained by the relatively small size of the vessel relative to the focal zone of the transducer (Maxwell et al. 2011), or by standing waves (Burgess et al. 2012).
19.3.1.2.2 Ultrasound Therapy with Microbubbles, but Without a Thrombolytic
Sonothrombolysis was investigated in several in-vitro (Borrelli et al. 2012) and in-vivo (Birnbaum et al. 1998; Culp et al. 2003; Xie et al. 2005) studies by insonation of microbubbles alone to initiate thrombolysis. These studies confirm thrombolytic efficacy using ultrasound and microbubbles alone, emphasizing the importance of cavitation activity. Several groups have developed thrombus-specific microbubbles with the ability to target clots for both imaging (Unger et al. 1998; Chen et al. 2009) and therapy (Alonso et al. 2009; Hagisawa et al. 2013) (Fig. 19.6).
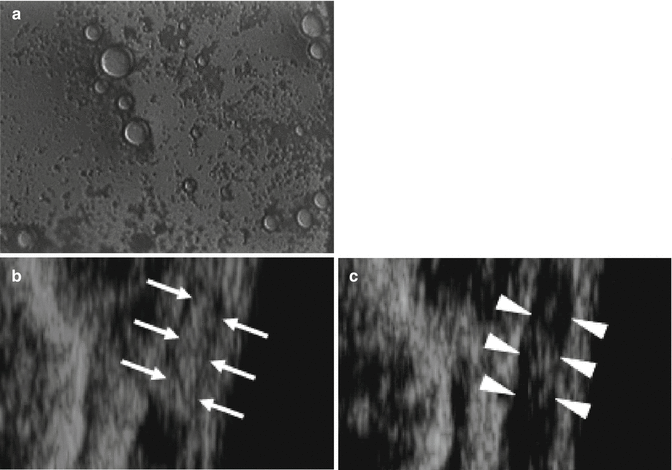
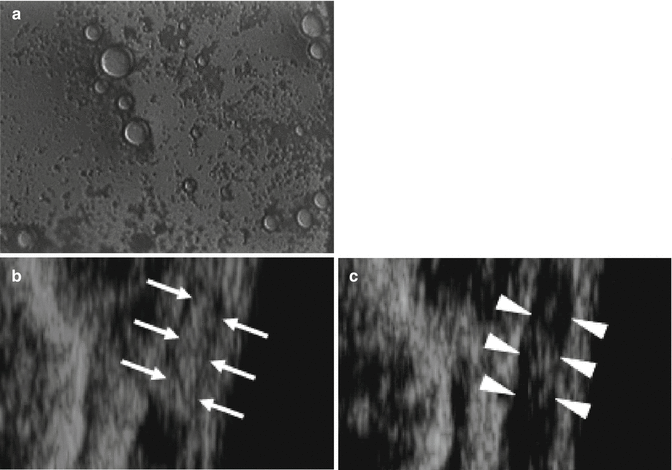
Fig. 19.6
(a) Photograph of thrombus-targeted microbubble bound to human whole blood clot in-vitro. Non-targeted microbubbles did not bind to the clot (Unger et al. 1998). (b) Thrombus-targeted bubble liposomes (white arrows) accumulate on thrombus within an occluded iliac artery in a rabbit model of thromboembolism. (c) After insonation of the thrombus-targeted bubble liposomes, flow was restored and the hyperechogenic area within the iliac artery was reduced (white arrowheads) (Hagisawa et al. 2013)
Culp (2004) investigated thrombolytic efficacy of a clot-targeted microbubble formulation in a porcine model. In a follow-up study (Culp et al. 2011), a rabbit carotid stroke model insonated with pulsed, 1-MHz ultrasound and microbubbles alone had a significantly smaller infarct volume than with rt-PA alone, or rt-PA with ultrasound exposure. However, there was no significant difference in the infarct volume if targeted or non-targeted microbubbles were used. Several additional studies demonstrated enhanced thrombolytic efficacy in-vitro (Unger et al. 1998; Chen et al. 2009) and in-vivo (Alonso et al. 2009; Hagisawa et al. 2013) using targeted microbubbles. It should be noted that the treatment parameters employed in several other studies did not produce appreciable thrombolytic efficacy with ultrasound alone (Frenkel et al. 2006; Datta et al. 2006; Prokop et al. 2007; Shaw et al. 2008; Petit et al. 2012a) or with microbubbles (Datta et al. 2008; Petit et al. 2012a; Bader et al. 2015).
19.3.1.2.3 Ultrasound Therapy with a Thrombolytic
Although lysis with ultrasound alone is a promising means to overcome strict contraindication criteria of thrombolytic drugs (Turi et al. 1993), drug-mediated thrombolysis remains the gold-standard in clinical practice. In this treatment regime, ultrasound is considered an adjuvant therapy, without the intent of completely replacing thrombolytic drugs. Early in-vitro studies by Lauer et al. (1992), and later by Francis and his colleagues (Francis et al. 1992; Blinc et al. 1993; Francis et al. 1995), found enhancement of rt-PA with ultrasound exposure. Meunier et al. (2007) found an increasing thrombolytic efficacy over rt-PA alone as a function of duty cycle over the range 10–80% at 120 kHz. However, subsequent studies by the same group found no distinct trend with duty cycle (Holland et al. 2008). Datta et al. (2006) demonstrated thrombolytic enhancement only when cavitation emissions were detected.
19.3.1.2.4 Ultrasound Therapy with Microbubbles and a Thrombolytic
Introducing ultrasound contrast agents, or stabilized microbubbles, can reduce the threshold for cavitation activity. De Saint et al. (2014) provides an extensive review of the use of microbubbles in sonothrombolysis. Tachibana and Tachibana (1995) pioneered the use of microbubbles in sonothrombolysis, insonating Albunex® with urokinase at 170 kHz to increase thrombolytic efficacy over urokinase alone, or urokinase with ultrasound exposure. Subsequent studies confirmed the thrombolytic enhancement in-vitro, with sub-megahertz ultrasound exposure (Datta et al. 2008; Hitchcock et al. 2011; Bader et al. 2015) as well as with diagnostic imaging frequencies (Kondo et al. 1999; Cintas et al. 2004; Xie et al. 2011). Nedelmann et al. (2010) found an increase in thrombolytic efficacy in-vivo with transcranial color-coded duplex Doppler, SonoVue®, and rt-PA when compared with rt-PA alone. Edema and lesion volumes were significantly smaller when microbubbles and rt-PA were exposed to ultrasound than exposed to rt-PA alone. However, Brown et al. (2011) found the infarct volume in a rabbit carotid artery model was not significantly smaller when Definity® and rt-PA were insonified compared to rt-PA alone. Hitchcock et al. (2011) developed a novel ex-vivo porcine carotid model and measured significant thrombolytic efficacy when Definity® and rt-PA were exposed to sub-megahertz ultrasound over rt-PA alone. Sutton et al. (2013b) extended this model, and showed that increased thrombolysis occurred only in unretracted clots. Xie et al. (2013) noted that epicardial recanalization was greatest in a porcine atherosclerotic model when Doppler pulses induced cavitation activity from Definity® in the presence of rt-PA.
Drug-loaded microbubbles also show potential as a “theragnostic” approach for enhancing thrombolytic efficacy. Echogenic liposomes (ELIP), liposomes containing air-filled microbubbles, can be used as a vector for therapeutic drugs. Incorporation of rt-PA into ELIP, or t-ELIP, allows acoustic activation (Smith et al. 2010) for localized drug delivery. In-vitro studies have demonstrated enhanced thrombolytic efficacy of t-ELIP and ultrasound over rt-PA alone (Shaw et al. 2009), or over rt-PA, ultrasound and OptisonTM combined (Tiukinhoy-Laing et al. 2007). Hua et al. (2014) treated thrombi in a rabbit femoral artery using targeted microbubbles loaded with rt-PA and 2-MHz ultrasound. The recanalization rates obtained with rt-PA targeted microbubbles were similar to those with a combination of untargeted microbubbles and free rt-PA.
19.3.1.3 Conclusions of Bench Top Studies
Ultrasound is well established to enhance thrombolysis in-vitro (Datta et al. 2006; Prokop et al. 2007), ex-vivo (Hitchcock et al. 2011), and in-vivo (Culp 2004; Xie et al. 2013). Mechanical effects, particularly acoustic cavitation (Petit et al. 2012a; Wu et al. 2014; Bader et al. 2015), are clearly responsible for enhanced thrombolysis. These bench top experiments provide proof of concept for a particular insonation scheme, but the results are restricted to the particular clot model employed (Xie et al. 2011). Given the variability in thrombi composition in-vivo (Liebeskind et al. 2011), future studies should focus on both the insonation regime and subtype of clot in order to employ the optimal treatment regime. For example, thrombolytic enhancement was not possible for low-amplitude (<0.25 MPa peak negative pressure), sub-megahertz insonation of retracted clots treated with Definity® and rt-PA (Sutton et al. 2013b). Other insonation schemes need to be developed for these stiff, retracted thrombi. Studies are also needed to determine the thrombus type, for example using elastography techniques (Viola et al. 2004) to determine the optimal ultrasound approach. Finally, methods to monitor and quantify the dose of cavitation energy, possibly employing PCI (Haworth et al. 2012), need to be further developed in order to monitor treatment progress.
19.3.2 Clinical Trials
19.3.2.1 Catheter-Directed Thrombolysis
Significant progress has been made over the past decade to translate ultrasound from the bench to the bedside. Catheter-directed ultrasound-accelerated thrombolysis has been successfully employed to treat peripheral arterial occlusions (Greenberg et al. 1999), stroke (The IMS II Trial Investigators 2007), deep venous thrombosis (Raabe 2006), and pulmonary embolism (PE) (Engelberger and Kucher 2014). Catheter-based ultrasound initiated complete or partial recanalization in 87.9% of cases in a review of 340 clinical cases (Doomernik et al. 2011). Overall rates of non-response (8.2%), complications (7.1%), bleeding (4.1%) and re-occlusion (2.1%) were low compared to conventional thrombolytic treatment. No distal embolization was reported during treatment. Engelberger and Kucher (2014) compiled the results from clinical studies of PE treatment with ultrasound catheter-directed thrombolysis and found similar clinical outcomes compared to administration of the thrombolytic tenecteplase, a genetically modified version of rt-PA (Baruah et al. 2006). In the first randomized trial employing an ultrasound catheter device, 59 patients with acute PE were treated with either the EKOS Endovascular System and rt-PA, or anticoagulation therapy alone (Kucher et al. 2013). The authors concluded ultrasound-assisted thrombolysis was the superior treatment versus anticoagulation at 24 h, without an increased bleeding risk.
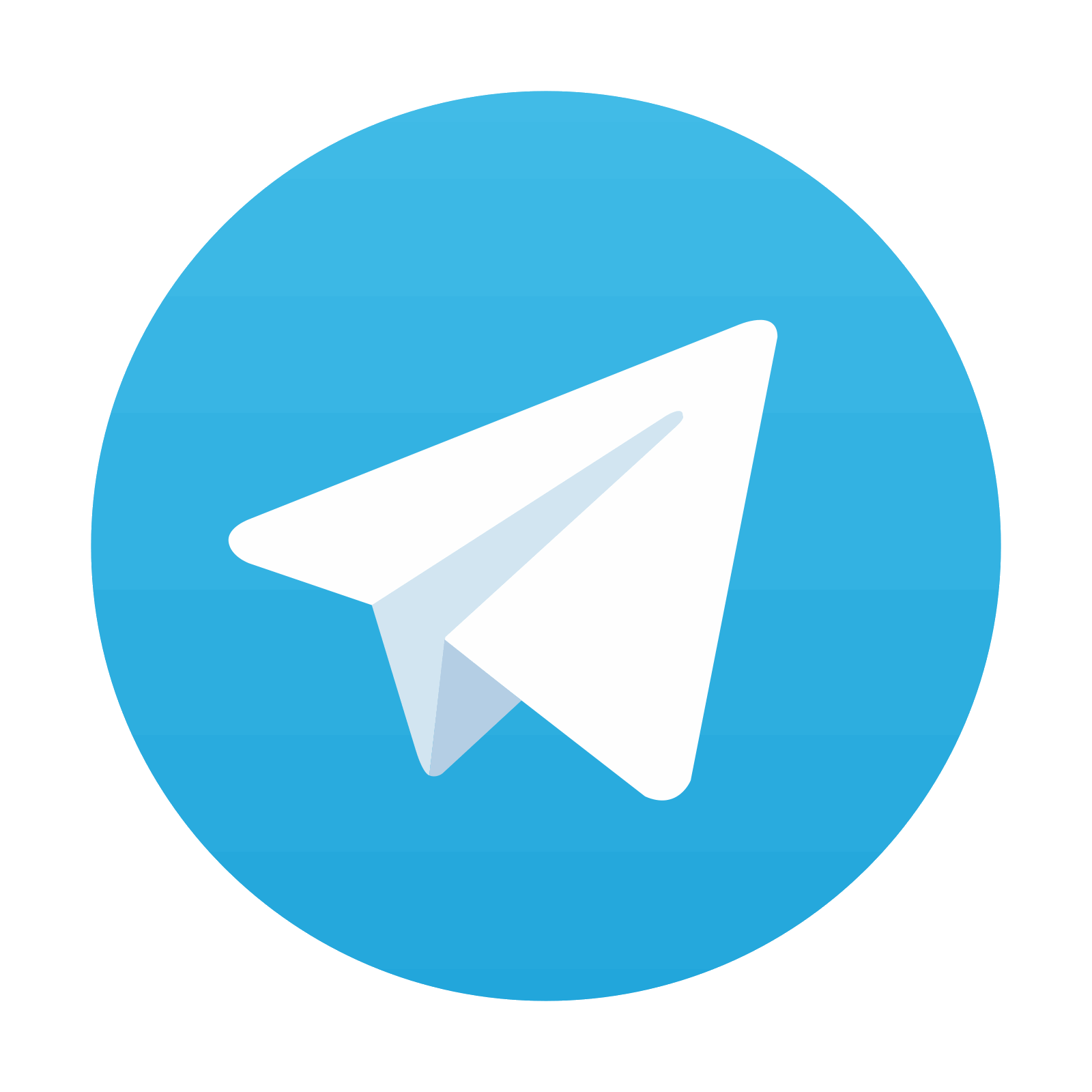
Stay updated, free articles. Join our Telegram channel
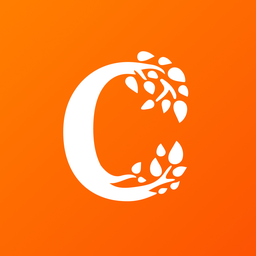
Full access? Get Clinical Tree
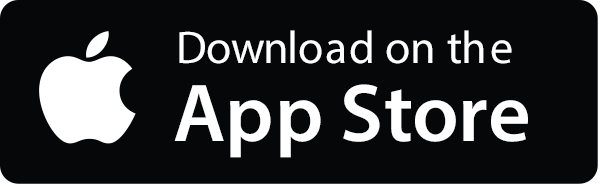
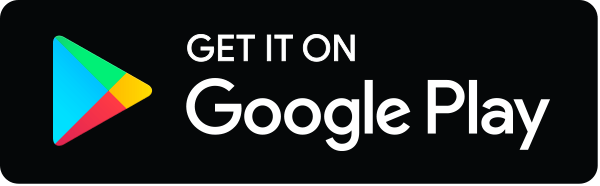