The Early History of Arterial Spin Labeling Magnetic Resonance Imaging: 1992 to 2000
John A. Detre
Arterial spin labeled (ASL) perfusion magnetic resonance imaging (MRI) primarily developed from a desire to exploit noninvasive magnetic resonance methods for use in monitoring brain function because neural activity is closely coupled with regional cerebral blood flow (CBF) and metabolism.1 Most functional imaging methods rely on this phenomenon.
As an undergraduate and medical student at Yale University in the early 1980s, I became interested in brain research and had the opportunity to work in the laboratory of Paul Greengard, who subsequently shared the Nobel Prize in Physiology and Medicine in 2000 for his work on signal transduction in the nervous system. In the Greengard lab, I studied cyclic guanosine monophosphate-dependent protein phosphorylation, mostly using polyacrylamide gel electrophoresis and autoradiography to measure phosphorylation in brain extracts incubated with 32P. Although this approach was powerful, I really wanted to be able to study brain function, ideally human brain function, in vivo.
In 1983, I attended a seminar at Yale by John Gore about noninvasive imaging and spectroscopy using nuclear magnetic resonance (NMR) and immediately knew that this was something I wanted to pursue. Although John Gore had been involved in early clinical imaging at Hammersmith Hospital in London before coming to Yale, much of the excitement for in vivo MRI centered around the use of 31P-NMR to measure adenosine triphosphate (ATP), the energy currency of living tissues. With the help of Jim Pritchard, I arranged to spend most of my fourth year of medical school in London working in David Gadian’s laboratory at the Royal College of Surgeons. Gadian was a pioneer of in vivo NMR and was doing in vivo 31P-NMR studies in rodent models.
After completing medical school at Yale and a year of internship at the University of Pittsburgh, I joined the Pittsburgh NMR Center for Biomedical Research at Carnegie Mellon University, working under Chien Ho and Alan Koretsky. Alan shared an interest in studying brain function in vivo, and we set out to use 31P-NMR to measure changes in brain function with pharmacologic stimulation. Despite a lot of prior success using 31P-NMR to study muscle function in both health and disease, spectroscopic measurements of ATP levels in the brain did not appear to be able to measure even marked changes in brain metabolism, likely because ATP levels were more tightly regulated in the brain than in skeletal or cardiac muscle.2 It ultimately required a spectroscopic measurement of CBF to detect the effects of a relatively large dose of amphetamine on the rat brain. For those studies, we used 19F-NMR to measure the washout of trifluoromethane and quantify CBF, as previously reported by Eleff et al.3 at the University of Pennsylvania. The washout kinetics of a diffusible tracer provide a direct measurement of tissue blood flow, which is proportional to the rate constant for the observed exponential decay of the tracer. Tracer washout was also the basis for perfusion imaging based on 15O-PET, and this derives from the seminal work of Kety and Schmitt,4 who originally used nitrous oxide as a perfusion tracer.
For personal reasons, I wound up moving to the University of Pennsylvania shortly thereafter and joined Jack Leigh’s laboratory to further pursue the development of MRI methods of measuring CBF as a biomarker of brain function. Another approach for measuring tissue perfusion with NMR used 2H2O (deuterated water) along with 2H NMR5 to measure the washout of 2H2O directly injected into tumor tissue. Working with David Smith, an anesthesiologist at University of Pennsylvania, the washout kinetics approach was also extended to an intra-arterial injection of deuterated saline to provide the first MRI of CBF, obtained in cat brain.6 Neither the 19F nor the 2H approach was ideally suited for noninvasive human applications because of the need to administer these exogenous tracers.
Perfusion and related parameters can also be inferred from the dynamic passage of nondiffusible (intravascular) tracers,7 and dynamic susceptibility contrast MRI based on approved MRI contrast agents has become a standard method for measuring perfusion in the clinic. Blood volume measurements using this approach were also applied to functional MRI (fMRI), making the cover of Science magazine,8 but were ultimately poorly suited for fMRI because of limitations in tracer dosing, and this method was rapidly eclipsed by blood oxygenation level–dependent (BOLD) fMRI.
The concept of ASL perfusion MRI was adapted from existing 15O-PET methodologies but was based on a “steady state” rather than the “dynamic” method that had been used in prior magnetic resonance measurements of diffusible tracers. In the steady state approach, instead of monitoring tracer kinetics dynamically, a constant delivery of tracer owing to CBF is balanced against its radioactive decay to relate positron emission tomography (PET) images of 15O-water concentration to CBF.9 In ASL, T1 relaxation replaces radioactive decay after magnetic labeling turns arterial blood water into a completely noninvasive endogenous tracer. Because T1 relaxation is relatively short, approximately 100 times faster than the radioactive
decay rate of 15O, only a small amount of labeled water accumulates. This subtle difference between images acquired with and without ASL can be measured and modeled to derive a calculated blood flow image showing perfusion in milliliters per gram per minute at each voxel.
decay rate of 15O, only a small amount of labeled water accumulates. This subtle difference between images acquired with and without ASL can be measured and modeled to derive a calculated blood flow image showing perfusion in milliliters per gram per minute at each voxel.
The idea of using magnetically labeled arterial water as a T1-based tracer was derived from my discussions with Jack Leigh after reading a chapter describing the steady state PET method in a book about cerebral blood flow.10 The first demonstration of ASL perfusion MRI was carried out in rat brain at 4.7T in collaboration with Alan Koretsky and Don Williams at the Pittsburgh NMR Center, where I was continuing to carry out experiments for approximately 1 week per month. Our first attempt used pseudo-continuous saturation of a 1-cm slab in the rat’s neck to magnetically label proximal arterial blood. The first experiment was successful, showing a clear signal difference between labeling proximally in the neck versus distally outside the brain, and this signal difference disappeared after the rat was sacrificed.11 Cerebral blood flow was obtained from the control/label difference by modifying the Bloch equation for Z magnetization to include flow terms, a model that was derived from Alan’s prior work in saturation transfer spectroscopy. The resulting images were crude, and it took almost 2 years to get this work published. By that time, the next iteration of ASL, using velocity-driven adiabatic fast passage, adapted from angiography12 to increase the labeling efficiency, had greatly improved the quality of continuous ASL (CASL) perfusion images of the rat brain.13
The first ASL experiments were actually contemporaneous with the first BOLD fMRI experiments, but because of its relative methodologic complexities, and perhaps also the lack of an equally compelling acronym, ASL was much slower to gain widespread recognition. Although BOLD MRI measures CBF changes indirectly through its effects on regional deoxyhemoglobin levels, ASL MRI measures CBF directly and provides absolute quantification of CBF in classical perfusion units of milliliters per gram per minute with appropriate modeling and assumptions. One of the earliest studies to demonstrate BOLD contrast changes with task activation also showed activation changes on inversion recovery imaging that were attributed to effects of ASL.14
The identical approach to that in the Williams et al.13 article was subsequently implemented for human scanning at 1.5T by Dave Roberts, then a graduate student.15,16 But the image quality obtained in human brain was much worse than in rat brain because T1 relaxation is faster at lower field strength, resulting in reduced accumulation of spin label in the brain. Because transit times from the labeling location to the tissue are much longer in humans than in rats, a significant amount of residual label concentrated in arteries, which produced bright signals now termed transit artifact. The recognition that this artifact could be reduced through the introduction of a delay between labeling and imaging and the corresponding two-compartment (blood and tissue) model for quantifying CBF were major advances leading to high-quality human CBF images with ASL, contributed by Alsop and Detre.17 In parallel studies at the National Institutes of Health, Ye et al.18 demonstrated that transit artifact could be attenuated by vascular crushing gradients and that relaxation of the spin label during arterial transit was a confound in ASL quantification. Their approach was successfully validated against 15O-PET CBF by Ye et al.19 at the National Institutes of Health. Although arterial transit effects are potential sources of artifact in ASL MRI data, arterial transit times can also be measured by ASL18,20 and likely have physiologic relevance.
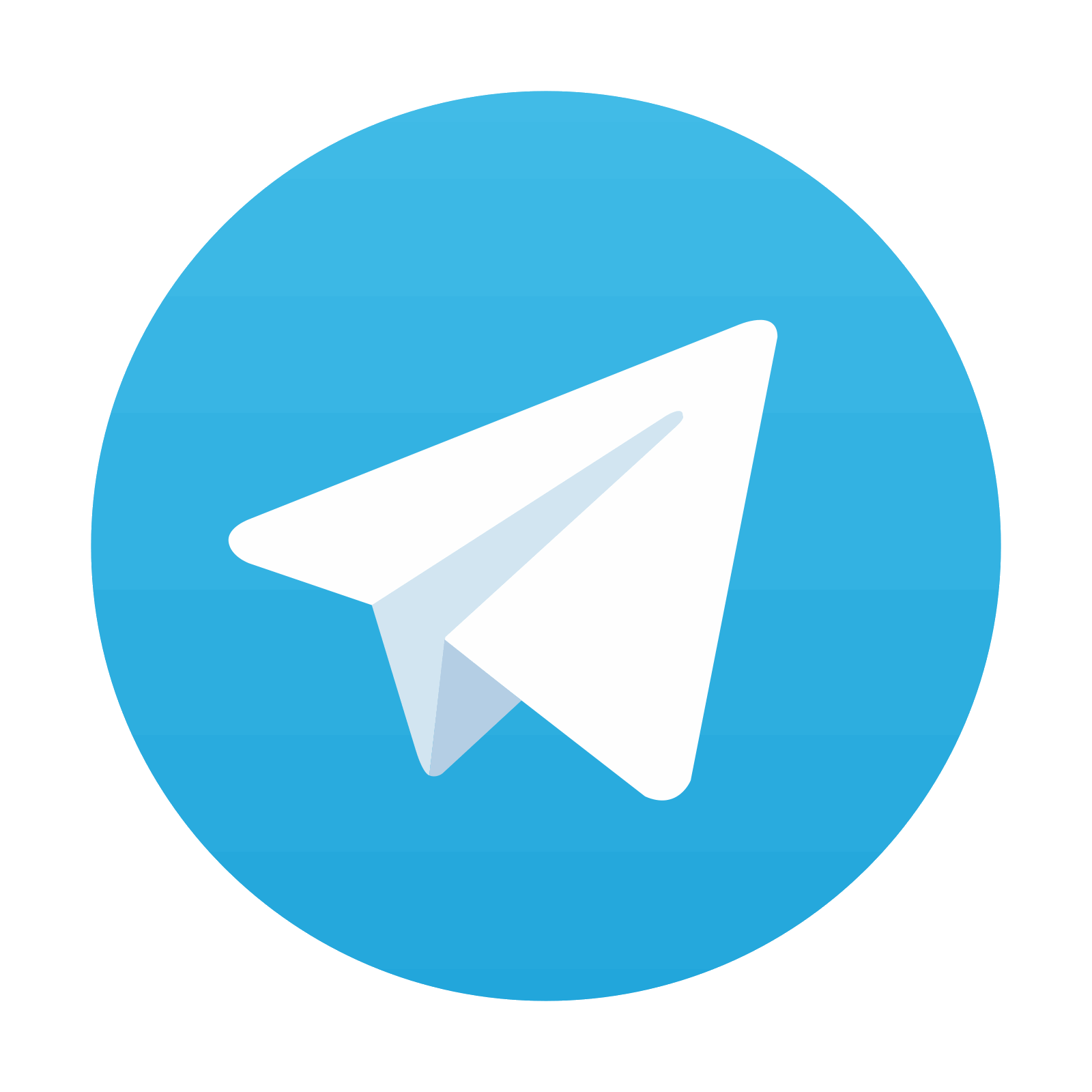
Stay updated, free articles. Join our Telegram channel
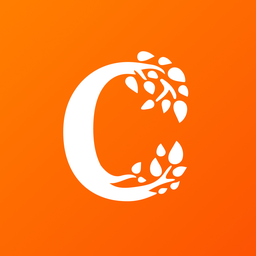
Full access? Get Clinical Tree
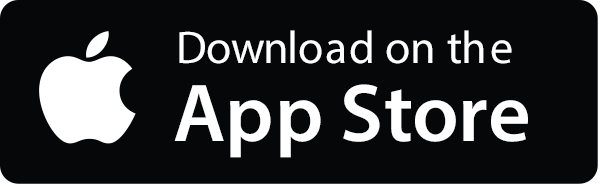
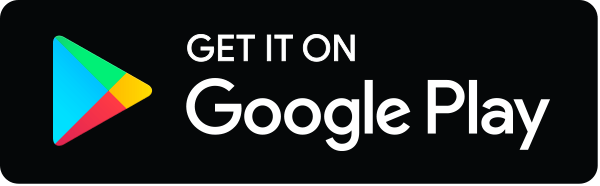