The Role of Multimodal Brain Imaging in Clinical Stroke Trials
Bruce C.V. Campbell
Geoffrey A. Donnan
Stephen M. Davis
Neuroimaging has revolutionized the clinical care of stroke by allowing differentiation of ischemia from hemorrhage and offering insights into individual pathophysiology. Imaging has also been used in clinical trials both to select patients for therapies and to monitor the outcomes of those therapies with varying success. This chapter discusses the principles of imaging selection and surrogate outcomes, the published and ongoing trials to date, and lessons that can be learned for future studies.
Selecting Patients for Reperfusion Therapies with Maximum Potential Benefit and Minimum Risk
Stroke pathophysiology is extremely heterogeneous between individuals. When an artery is occluded, there is a varying capacity for alternative supply vessels called collaterals to maintain tissue viability in the affected vascular territory for a period of time. The brain region that is nonfunctional but potentially salvageable with rapid reperfusion is termed the ischemic penumbra. The irreversibly injured region is termed the ischemic core. Some patients have good collateral flow, others poor collaterals, and some patients spontaneously reperfuse. These variables have a major impact on the volume of ischemic penumbra downstream of an arterial occlusion and interact with time from onset. The potential clinical response to reperfusion therapies is directly related to the volume and location of ischemic penumbra versus irreversibly injured ischemic core.
All the phase 3 trials of tissue plasminogen activator (tPA) to date have been based on clinical criteria and noncontrast CT selection to exclude hemorrhage and major established infarction.1 Such trial designs have, as yet, been unable to demonstrate benefit of thrombolysis beyond 4.5 hours, despite strong evidence of persisting ischemic penumbra in a proportion of patients. The promise of imaging selection has been to identify those patients with a large penumbra and small core, so they can be targeted for therapy. Selecting patients with a small ischemic core is important; a large ischemic core is one of the strongest predictors of poor clinical outcome, and reperfusion has very little chance of translating into a good clinical outcome.2,3,4 This is especially important in phase 3 trials using a modified Rankin scale (mRS) 0–1 outcome, which is very unlikely to be achieved if the baseline core volume is large (>50 to 70 mL). At the opposite end of the spectrum, patients with a small core in a small perfusion lesion are also unlikely to positively contribute to a clinical trial as they are very likely to do well without treatment, and so there is a limit on the maximum potential benefit to be gained from reperfusion. If the patient has already spontaneously reperfused, he or she presumably has nothing to gain from therapies aimed at inducing reperfusion. Such patients will also dilute the observed treatment effect in any trial.
By reducing heterogeneity, imaging selection should allow a reduction in the required sample size to demonstrate an effect. This chapter will also discuss the potential for imaging selection to reduce the risk of hemorrhagic transformation, the major risk of reperfusion therapies. Although time to treatment has not been significantly associated with increased hemorrhagic transformation, this is one of the potential contributors to the lack of reperfusion benefit in noncontrast computed tomography (CT) selected patients beyond 4.5 hours.
Perfusion imaging in acute stroke trials is the major theme of this chapter, but it cannot be considered in isolation from other parameters that are an essential part of the use of multimodal magnetic resonance imaging (MRI) in the acute stroke setting. Therefore, the discussion here will be broadened to include imaging selection approaches in general.
Current Algorithms for Treatment Selection
The field of stroke imaging has been criticized for a lack of standardization in approach and in the specifics of parameters and thresholds chosen.5,6 The resultant heterogeneity has complicated the assessment of different treatment selection approaches.
Within 4.5 hours, most groups advocate the quick, simple, and proven noncontrast CT-based approach. Multimodal imaging, including perfusion in many centers, has been largely used to improve diagnostic confidence and for selection into trials. Elsewhere, imaging selection has been applied in clinical practice to select patients for treatment beyond 4.5 hours. In Germany, there is extensive published experience with qualitatively assessed perfusion–diffusion mismatch.7,8 This appears to have reasonable safety in uncontrolled case series. Similar approaches have been applied more widely in Europe and North America. Other proposed paradigms include MR angiography–diffusion mismatch, which infers the presence of salvageable penumbra when there is a large vessel occlusion and a small diffusion lesion.9 This has the potential advantage of
avoiding the need for contrast and the perceived “complicated” interpretation of perfusion maps. Clinical-diffusion mismatch is another proposed method of identifying patients with a large, nonfunctioning penumbra by selecting patients with a severe clinical deficit out of proportion to the small irreversibly injured ischemic core.10 This seems to have moderate sensitivity compared with perfusion–diffusion mismatch. More quantitative diffusion–perfusion mismatch has, until recently, been restricted to offline analysis in trials due to the lack of automated software. Quantitative mismatch generally uses both relative (ratio) and absolute volume criteria. The relative criterion prevents overestimating rates of mismatch in large lesions where a small rim of hypoperfusion around the core can have a large volume. The absolute volume criterion avoids overestimating rates of mismatch in small core lesions where a slightly larger, but still clinically insignificant, volume of perfusion lesion could give a high mismatch ratio. The mismatch ratio used in the EchoPlanar Imaging Thrombolytic Evaluation Trial (EPITHET) and Diffusion and Perfusion Imaging Evaluation for Understanding Stroke Evolution (DEFUSE) studies of greater than 1.2 was based on the minimum visually recognizable volume difference, but others have used higher thresholds. An additional criterion for “target mismatch” in more recent studies is a maximum ischemic core volume. Undoubtedly, larger ischemic core volume is associated with poorer outcome, but this is heavily influenced by the eloquence of the affected region. Even patients with large cores may benefit from reperfusion-induced salvage of peripheral regions, although they may not attain the mRS 0–1 outcome often required in trials. Whether ischemic core volume should be capped at 100 mL,11 70 mL,2 or 25 mL3 in trials is a balance between increased treatment response with smaller cores versus lower recruitment rates and potentially trial generalizability. The choice also depends on the level of functional outcome classified as treatment success (e.g., mRS 0–3 would allow enrollment of patients with larger ischemic core volumes than mRS 0–1).
avoiding the need for contrast and the perceived “complicated” interpretation of perfusion maps. Clinical-diffusion mismatch is another proposed method of identifying patients with a large, nonfunctioning penumbra by selecting patients with a severe clinical deficit out of proportion to the small irreversibly injured ischemic core.10 This seems to have moderate sensitivity compared with perfusion–diffusion mismatch. More quantitative diffusion–perfusion mismatch has, until recently, been restricted to offline analysis in trials due to the lack of automated software. Quantitative mismatch generally uses both relative (ratio) and absolute volume criteria. The relative criterion prevents overestimating rates of mismatch in large lesions where a small rim of hypoperfusion around the core can have a large volume. The absolute volume criterion avoids overestimating rates of mismatch in small core lesions where a slightly larger, but still clinically insignificant, volume of perfusion lesion could give a high mismatch ratio. The mismatch ratio used in the EchoPlanar Imaging Thrombolytic Evaluation Trial (EPITHET) and Diffusion and Perfusion Imaging Evaluation for Understanding Stroke Evolution (DEFUSE) studies of greater than 1.2 was based on the minimum visually recognizable volume difference, but others have used higher thresholds. An additional criterion for “target mismatch” in more recent studies is a maximum ischemic core volume. Undoubtedly, larger ischemic core volume is associated with poorer outcome, but this is heavily influenced by the eloquence of the affected region. Even patients with large cores may benefit from reperfusion-induced salvage of peripheral regions, although they may not attain the mRS 0–1 outcome often required in trials. Whether ischemic core volume should be capped at 100 mL,11 70 mL,2 or 25 mL3 in trials is a balance between increased treatment response with smaller cores versus lower recruitment rates and potentially trial generalizability. The choice also depends on the level of functional outcome classified as treatment success (e.g., mRS 0–3 would allow enrollment of patients with larger ischemic core volumes than mRS 0–1).
The philosophy underlying the imaging-based selection described has generally been driven by a desire to move away from rigid arbitrary time windows toward tissue-based pathophysiologic selection. However, some authors have used the absence of T2-weighted fluid-attenuated inversion recovery (FLAIR) hyperintensity as an indication that patients are within the “treatable” 4.5-hour thrombolysis window when onset time is uncertain.12 Apart from this form of imaging selection being somewhat contrary to the above philosophy, many patients within the conventional 4.5-hour treatment window have established T2-weighted FLAIR hyperintensity.13,14,15 Furthermore, there is no evidence that patients with T2-weighted FLAIR hyperintense infarcts differ in their response to tPA.16 Although those few patients who are FLAIR negative will probably do well with treatment, this strategy may be excluding patients who might otherwise benefit from reperfusion. Two large randomized trials (WAKE-UP [efficacy and safety of MRI-based thrombolysis in wake-up stroke] in Germany and MR-WITNESS [multi-center safety trial of IV rt-PA in patients with unwitnessed stroke onset] in the United States) are ongoing based on the FLAIR “tissue clock” approach in uncertain-onset stroke.
The Mismatch Paradigm: Magnetic Resonance versus Computed Tomography
Perfusion-Diffusion Mismatch
Using MRI, diffusion-weighted imaging (DWI) is generally a clinically reliable method to define irreversible injury. Although there have been varying reports of diffusion lesion reversal,17,18 many of these were confounded by infarct atrophy or unrecognized temporary reversal of diffusion lesions immediately after reperfusion.19 Although very early reperfusion may, in the future, be demonstrated to lead to clinically relevant diffusion reversal, for current clinical purposes the diffusion lesion can be regarded as an accurate reflection of ischemic core, and adjusting for possible reversal rarely alters mismatch status.20,21 The main challenge in perfusion-diffusion mismatch is, therefore, the definition of the perfusion lesion to distinguish tissue at risk of infarction from benign oligemia (tissue that will not infarct even in the absence of reperfusion). Two main approaches have been followed. The first was to define “penumbra” as tissue destined to infarct in the absence of reperfusion. Using a receiver operating characteristic–based methodology, Christensen et al.22,23 concluded that a time to maximum (Tmax) threshold of approximately 5 to 6 seconds was the optimal threshold. The second strategy was to compare MRI perfusion to contemporaneous positron emission tomography imaging and use the well-validated 20 mL/100 g/min positron emission tomography—cerebral blood flow (CBF) threshold to define penumbra. Again, Tmax appeared a robust parameter for this purpose and a threshold of 5.5 seconds was optimal in receiver operating characteristic analysis.24,25 The major stroke imaging–based trials (EPITHET, DEFUSE, DEFUSE-2, MR-RESCUE and the Extending the Time for Thrombolysis in Emergency Neurological Deficits [EXTEND]) have all used Tmax.
The thresholds presented above are clearly averages, and the real threshold that best matches tissue destined to infarct in the absence of reperfusion can vary markedly between patients. An alternative approach has been suggested based on probabilities of infarction at each level of Tmax delay.26 However, even this approach does not circumvent the snapshot nature of the information provided by perfusion imaging. Fluctuations in collateral flow over time may well be contributing to the unpredictable nature of infarct growth.
Volumetric mismatch has traditionally been calculated as perfusion lesion volume—diffusion lesion volume
without reference to spatial overlap. However, clot migration or fragmentation can sometimes lead to a diffusion lesion outside the perfusion lesion, and, therefore, an underestimation of the true volume of tissue at risk of infarction.27 This is increasingly common as time passes.28 Coregistration of diffusion and perfusion images, so that only a diffusion lesion within the perfusion lesion is considered in mismatch calculations can account for this spatial topography and increases the proportion of patients with mismatch. A reanalysis of the EPITHET study using coregistered mismatch led to a positive result.29 Nevertheless, echoplanar image distortion and suboptimal data quality due to motion in acutely unwell stroke patients can often prevent accurate coregistration. Any inaccuracy in coregistration artificially inflates the apparent volume of coregistered mismatch. This needs to be carefully scrutinized if a coregistered approach is to be used. Contemporary trials such as DEFUSE-2 and EXTEND, which use fully automated mismatch analysis, have, therefore, chosen not to use coregistered mismatch.
without reference to spatial overlap. However, clot migration or fragmentation can sometimes lead to a diffusion lesion outside the perfusion lesion, and, therefore, an underestimation of the true volume of tissue at risk of infarction.27 This is increasingly common as time passes.28 Coregistration of diffusion and perfusion images, so that only a diffusion lesion within the perfusion lesion is considered in mismatch calculations can account for this spatial topography and increases the proportion of patients with mismatch. A reanalysis of the EPITHET study using coregistered mismatch led to a positive result.29 Nevertheless, echoplanar image distortion and suboptimal data quality due to motion in acutely unwell stroke patients can often prevent accurate coregistration. Any inaccuracy in coregistration artificially inflates the apparent volume of coregistered mismatch. This needs to be carefully scrutinized if a coregistered approach is to be used. Contemporary trials such as DEFUSE-2 and EXTEND, which use fully automated mismatch analysis, have, therefore, chosen not to use coregistered mismatch.
An important caveat with the mismatch paradigm is topographical eloquence. The simple volume of tissue involved correlates strongly with outcome, but clearly some brain regions have more clinically evident functions than others. Consideration of where the irreversible versus salvageable tissue lies is a challenge for future refinements of mismatch selection.
Computed Tomography Perfusion
In the absence of diffusion MRI, the major challenge of CT perfusion mismatch is accurate identification of the ischemic core. One study suggested absolute cerebral blood volume (CBV) was the optimal parameter to define ischemic core with a threshold less than 2 g/100 mL.30 However, recent publications from multiple groups have found relative CBF performs better than absolute CBF or CBV.31,32,33 Unfortunately the thresholds required vary considerably between postprocessing approaches in different proprietary software packages. There is certainly imprecision in CT-CBF estimation of the ischemic core compared with diffusion MRI. The lower contrast-to-noise ratio of CT perfusion makes acquisition parameters, including bolus quality, critically important. Provided good quality data are acquired, the inaccuracies in ischemic core are mostly due to (a) large vessels (e.g., around the Sylvian fissure), which mask the local tissue status, and (b) leukoaraiosis, where CBF (and CBV) are often below the critical threshold. Tmax thresholds for “penumbra” appear to directly translate from MRI to CT perfusion (CTP).34
Computed Tomography versus Magnetic Resonance: Advantages and Disadvantages
There is no doubt that MRI has some advantages over CT: It is radiation free and DWI is significantly more precise than CTP–regional CBF (rCBF) in estimating ischemic core. Time-of-flight MR angiography is contrast-agent free, and arterial spin labeling (ASL) has promise as a contrast-agent free perfusion metric that can produce quantitative CBF measurements, thus avoiding current contraindications in patients with renal disease. Currently, however, accurate measurement of ASL CBF in stroke is limited by the degree of delay in collateral perfusion, which leads to signal dropout and falsely low CBF.35 The main contemporary limitations of MRI are limited emergency access and a range of contraindications (e.g., pacemakers, aortic stents), which can be difficult to assess in dysphasic or confused patients. Gadolinium contrast for MRI has a lower rate of allergic reactions than iodinated contrast used for CTP and is nonnephrotoxic. Nevertheless, in patients with renal failure, gadolinium is contraindicated due to the rare but potentially fatal complication of nephrogenic systemic fibrosis. In practice, up to 20% of patients may be unsuitable for MRI.
In current practice, most emergency departments in developed countries have immediate access to a CT scanner capable of CTP. Apart from reduced time to access the scanner, the acquisition of CT, CTP, or CT angiography is generally faster than a stroke MRI. This is particularly the case with current generation CT scanners capable of simultaneous dynamic CT angiography and CTP acquisitions. Despite some imprecision compared with MRI, largely relating to ischemic core, initial data suggest that mismatch-based treatment decisions rarely differ between CT and MRI.34 Previous technical limitations of brain coverage and acquisition duration are rapidly vanishing as older machines are replaced. Technical aspects of CT and MR perfusion acquisition are dealt with in other chapters, but examples of acute stroke protocols for multimodal CT and MR are described in Tables 45.1 and 45.2.
In summary, either modality is probably capable of accurate assessment at a decision-making level. In current practice, CT is generally easier and faster, but this may change if MRI becomes a routine modality in emergency departments and as MR-incompatible devices become obsolete.
Arterial Occlusion Plus Small Ischemic Core Paradigm
As mentioned previously, an alternative to the mismatch paradigm that does not require perfusion imaging is artery-diffusion mismatch. Clearly this only applies to MRI, so the caveats of limited access to MRI in many centers and MRI contraindications discussed previously apply. In the presence of major arterial occlusion, a small ischemic core almost always implies a mismatch. This approach may, therefore, be adequate when considering endovascular therapies that are restricted to major arteries. However, most noninvasive angiographic techniques
have a limited ability to resolve more distal arterial occlusion (e.g., beyond middle cerebral artery [MCA] M2 segment). Perfusion imaging clearly demonstrates the effects of these more distal occlusions and the downstream tissue status. Although the magnitude of benefit may be less than that achieved by reperfusion of an M1 MCA occlusion, the territory involved can still be clinically significant. Furthermore, for trials of pharmacologic lysis, these distal occlusions are much more likely to recanalize and therefore, lead to clinical benefit than a large proximal occlusion. The authors, therefore, argue that perfusion-based mismatch is a more generalizable treatment paradigm.
have a limited ability to resolve more distal arterial occlusion (e.g., beyond middle cerebral artery [MCA] M2 segment). Perfusion imaging clearly demonstrates the effects of these more distal occlusions and the downstream tissue status. Although the magnitude of benefit may be less than that achieved by reperfusion of an M1 MCA occlusion, the territory involved can still be clinically significant. Furthermore, for trials of pharmacologic lysis, these distal occlusions are much more likely to recanalize and therefore, lead to clinical benefit than a large proximal occlusion. The authors, therefore, argue that perfusion-based mismatch is a more generalizable treatment paradigm.
TABLE 45.1 EXAMPLES of MULTIMODAL COMPUTED TOMOGRAPHY PROTOCOLS FOR ACUTE ISCHEMIC STROKE | ||||||||||
---|---|---|---|---|---|---|---|---|---|---|
|
TABLE 45.2 EXAMPLE of A MULTIMODAL MAGNETIC RESONANCE IMAGING PROTOCOL FOR ACUTE ISCHEMIC STROKE | ||||||||||||||||||||||||
---|---|---|---|---|---|---|---|---|---|---|---|---|---|---|---|---|---|---|---|---|---|---|---|---|
|
Collaterals
Collateral circulation is the key to the existence of ischemic penumbra. Traditionally, assessment of collaterals has been performed using simple visual scales applied to catheter angiography. CT angiographic studies have also been graded using simple scales and, even with the challenges of the “snapshot” in time depiction of collateral flow, have demonstrated a clear association of good collaterals with reduced infarct volume and improved clinical outcomes. The recent proliferation of four-dimensional CT angiographic acquisitions promises to redefine this area of research.
The relationship of collaterals to perfusion imaging and mismatch has been less studied. The authors propose that the area of delayed time to peak or Tmax in the territory usually supplied by an occluded vessel is a direct representation of collateral flow. Areas within that region with reduced CBV or restricted diffusion represent tissue where collateral flow has been inadequate to sustain viability. Mismatch is, therefore, intimately entwined with collateral quality, and the ability to quantitate spatial and temporal parameters probably provides advantages over the standard visual collateral grading scales.36
Prediction of Hemorrhagic Transformation
Symptomatic hemorrhagic transformation is generally associated with poor outcomes, so reducing the risk would clearly benefit patients and trial selection. Perfusion imaging may have an important role in the prediction of hemorrhagic transformation. Severe hypoperfusion is thought to lead to blood–brain barrier permeability with subsequent risk of hemorrhage should reperfusion occur. Protocols to assess permeability using perfusion imaging have been developed and are currently being tested. The Hyperintense Acute ρeperfusion Marker (HARM) sign is one means of detecting blood–brain permeability, reflected in contrast extravasation into cerebrospinal fluid after reperfusion on a delayed T2-weighted FLAIR scan.37 However, one of the challenges of detecting increased permeability in stroke prior to reperfusion is that the most permeable regions are most likely those with the least contrast arrival. This may reduce the reliability of measurements. An alternative approach is to identify the areas with the most severe hypoperfusion.38,39 Very low cerebral blood volume (VLCBV) is a simple analysis method to identify regions with virtually no detectable contrast arrival. VLCBV has good sensitivity and specificity in predicting parenchymal hematoma and appears a stronger predictor than diffusion lesion volume and large volumes of severely delayed Tmax.40 Large severe Tmax hypoperfusion lesions in isolation have been associated with increased risk of
hemorrhagic transformation,41 particularly using CT perfusion42 as well as constituting one of the criteria for a “malignant profile,” which indicates more general poor outcome after reperfusion.4 The malignant profile concept was developed in the DEFUSE trials and aims to identify patients who do worse after reperfusion than would be expected from natural history. Both accelerated edema and hemorrhagic transformation relate to increased blood–brain barrier permeability and constitute the likely mechanisms of worse outcomes despite reperfusion. The hypoperfusion intensity information provided by VLCBV is complementary to the malignant profile, which focuses more on lesion volumes. VLCBV, therefore, appears to have a particular advantage in predicting hemorrhage risk in small to moderate-sized diffusion lesions.39,40
hemorrhagic transformation,41 particularly using CT perfusion42 as well as constituting one of the criteria for a “malignant profile,” which indicates more general poor outcome after reperfusion.4 The malignant profile concept was developed in the DEFUSE trials and aims to identify patients who do worse after reperfusion than would be expected from natural history. Both accelerated edema and hemorrhagic transformation relate to increased blood–brain barrier permeability and constitute the likely mechanisms of worse outcomes despite reperfusion. The hypoperfusion intensity information provided by VLCBV is complementary to the malignant profile, which focuses more on lesion volumes. VLCBV, therefore, appears to have a particular advantage in predicting hemorrhage risk in small to moderate-sized diffusion lesions.39,40
Monitoring Success of Therapies
Assessment of Reperfusion and Recanalization
Reperfusion is a key biomarker of treatment efficacy. In most cases, recanalization leads to reperfusion. Nevertheless, distal clot migration (which may be beyond the resolution of noninvasive angiographic modalities) and perhaps the purported “no-reflow phenomenon” (which may reflect capillary sludging or venous outflow resistance) limit concordance. A degree of “reperfusion” (if defined as reduction in perfusion lesion volume) can also occur without recanalization due to improvement in collateral circulation over time. Data have suggested that reperfusion is a more meaningful predictor of outcome than recanalization.43
The timing of reperfusion assessment has been variable in studies and is generally a compromise between biologic relevance and pragmatic logistical considerations. Reperfusion assessment should probably be performed within 24 hours because most studies suggest that reperfusion beyond 24 hours is of limited benefit. There are, however, exceptions when selected patients with a large mismatch have experienced neurologic improvement with reperfusion beyond 24 hours.44,45 The Stroke Imaging Roadmap consensus statement recommended 1 to 6 hours posttreatment, which is particularly targeted at assessing the efficacy of the treatment intervention versus spontaneous lysis. Several transcranial Doppler–based studies have examined the first 2 hours after treatment.46,47,48 The DEFUSE and DEFUSE-2 studies used 3 to 6 hours.11,49 The tenecteplase versus alteplase trial assessed reperfusion at 24 hours,50 and EPITHET assessed reperfusion at 3 to 5 days after treatment.51 In all cases, reperfusion was strongly associated with clinical benefit. Nevertheless, the spontaneous reperfusion rate of 26% in the EPITHET placebo group suggests that earlier imaging may have been more specific for treatment effect. The 3 to 6 hour assessment in DEFUSE was logistically challenging and may have missed clinically relevant reperfusion in a few patients. Benefits of later reperfusion in mismatch patients with good collateral flow have been demonstrated in other studies (e.g., the subgroup treated 8 to 12 hours after onset in DEFUSE-2).
The actual criteria for reperfusion using perfusion imaging have also varied. EPITHET and DEFUSE used dichotomous definitions (>90% in EPITHET vs. >30% in DEFUSE and >50% in DEFUSE-2). In the authors’ experience, most patients either have major reperfusion or very little, so the rates tend to be similar for definitions in the 50% to 90% range. An alternative approach was used in the tenecteplase versus alteplase study, where reperfusion between baseline CT and 24-hour MRI was assessed as a continuous variable.
In reality, reperfusion is an immensely powerful predictor of clinical outcomes, and every measure used in the studies mentioned has yielded positive results, despite the considerable variation and often small sample sizes.
Assessment of Infarct Growth
Final infarct volume and infarct growth are strongly correlated with clinical outcome and have been used as a surrogate outcome in stroke trials. Reduction in infarct growth represents the basic mechanism of action of reperfusion therapies. Some authors have criticized this surrogate approach and reported that 24-hour stroke severity (National Institutes of Health Stroke Scale [NIHSS]) is just as, if not more, predictive of later functional outcome as infarct volume and growth.52 This may be the case (especially given the eloquence of different brain regions). However, for early phase proof of concept studies, removing the random element of topographical eloquence by measuring infarct growth is a positive feature that reduces trial outcome heterogeneity.
![]() FIGURE 45.1. Evolution of ischemic stroke. DWI, diffusion-weighted imaging; FLAIR, fluid-attenuated inversion recovery. |
The time at which these parameters have been assessed has varied widely, and there are distinct advantages and disadvantages of the different options. The kinetics of infarct evolution are initial growth due to genuine expansion into new brain regions, followed by further growth due to edema over several days. Subsequently infarct atrophy occurs over a few weeks and is mostly complete by day 30 (Fig. 45.1). The infarct initially expands
into new territory, then enlarges in volume due to edema, followed by resolution of edema and atrophy.
into new territory, then enlarges in volume due to edema, followed by resolution of edema and atrophy.
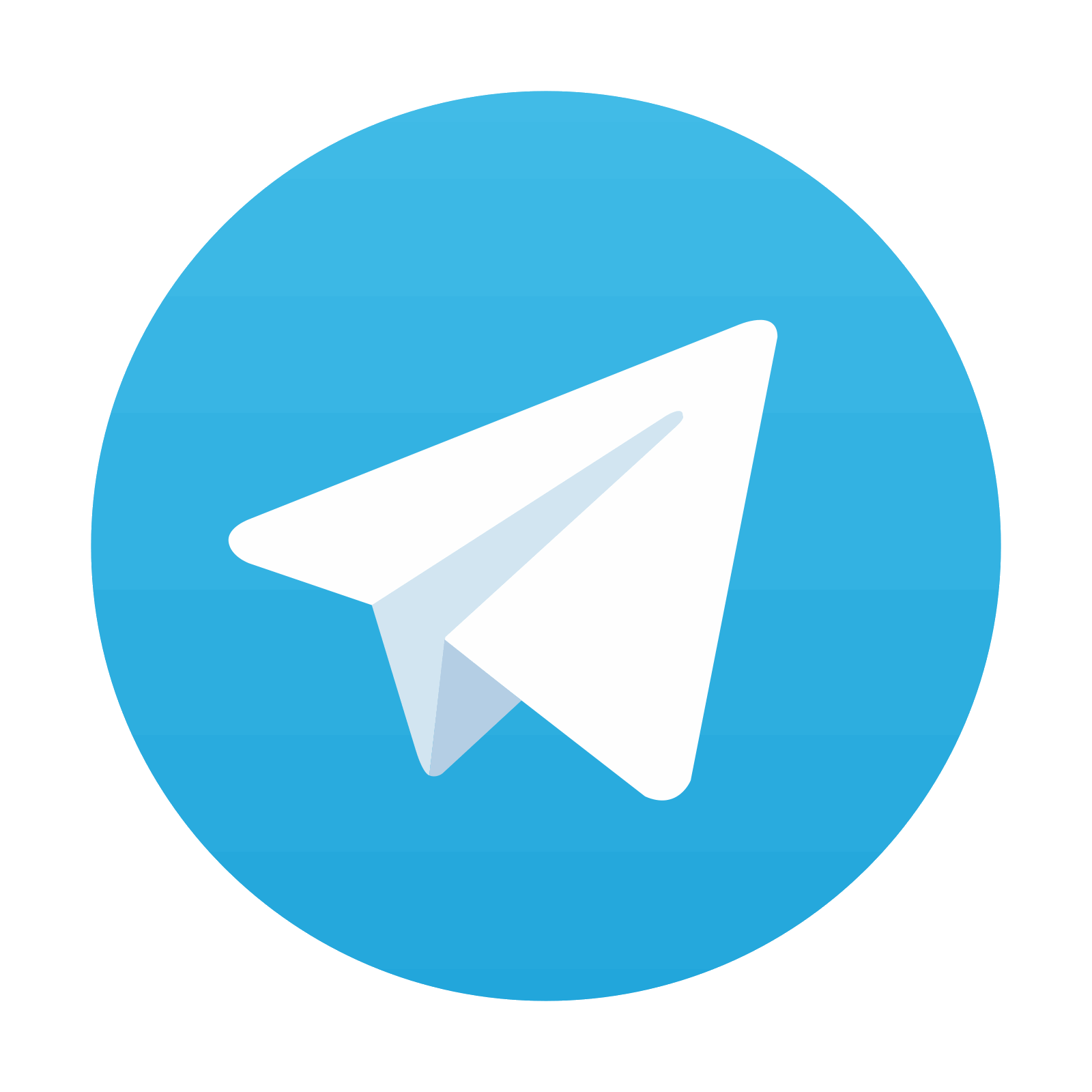
Stay updated, free articles. Join our Telegram channel
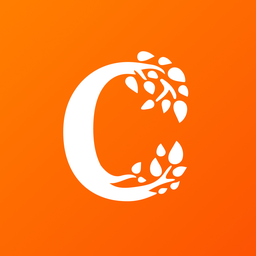
Full access? Get Clinical Tree
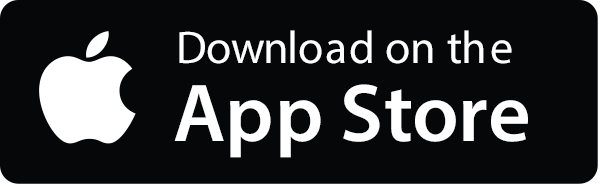
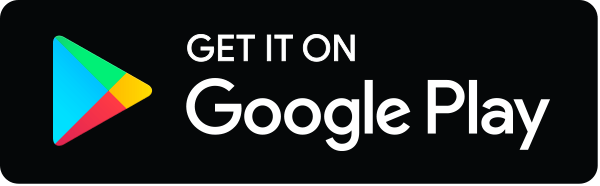