(1)
Department of Nuclear Medicine, Jacobi Medical Center, Bronx, NY, USA
7.1 Thyroid Anatomy
7.2.1 Iodide Transport
7.2.2 Hormone Synthesis
7.2.4 T3 and T4
7.2.5 Antithyroid Drugs
7.2.6 Summary
7.3.2 Iodine-123
7.3.3 Iodine-131
7.3.5 Summary
7.4.1 TSH Secretion
7.4.4 Summary
7.5.1 Iodine Deficiency
7.5.2 Iodine Excess
7.5.3 Summary
7.6 Endemic Goiter
7.6.1 Goitrogens
7.6.2 Pathophysiology
7.6.3 Radionuclide Procedures
7.6.4 Summary
7.7.1 Postpartum Thyroiditis
7.7.2 Viral Thyroiditis
7.7.4 Radionuclide Procedures
7.7.5 Summary
7.8.1 Etiological Factors
7.8.2 Pathophysiology
7.8.3 Radionuclide Procedures
7.8.4 Summary
7.9.1 Pathophysiology
7.9.3 Summary
7.10.1 Hyperthyroidism
7.10.2 Hypothyroidism
7.10.3 Summary
7.1 Thyroid Anatomy
The thyroid gland develops from the foramen cecum of the tongue, to which it is connected by the thyroglossal duct. It descends during fetal life to reach the anterior neck by about the seventh week, and absent or aberrant descent results in ectopic locations, including the sublingual region and superior mediastinum (Fig. 7.1). The thyroglossal duct undergoes atrophy, though remnant duct tissue frequently is visualized by scintigraphy as an upper midline neck structure following thyroidectomy and TSH stimulation. The duct remnant occasionally may form a cyst.
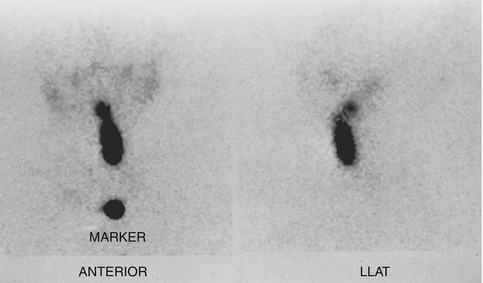
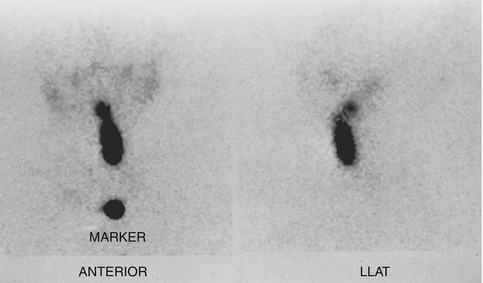
Fig. 7.1
Scintigraphic images in the anterior and left lateral projections show partly descended thyroid gland extending from the sublingual region to the upper neck
The normal adult thyroid gland in iodine-sufficient regions weighs about 14–18 g. It is generally smaller in women than in men and is barely palpable [1, 2]. The thyroid is located in the mid to lower anterior neck, with the isthmus in front of the trachea, usually just below the cricoid cartilage, and the lobes on the sides of the trachea. In older individuals with shorter necks, the thyroid may lie at or just above the suprasternal notch, and it is often partly substernal. The thyroid gland moves cephalad during swallowing, a characteristic that aids in palpation and in distinction of the thyroid from non-thyroid neck masses.
7.2 Hormone Synthesis and Secretion
7.2.1 Iodide Transport
The thyroid follicle consists of a colloid center, which acts as a storage site for thyroid hormone, surrounded by epithelial cells. The thyroid epithelial cell has a transport mechanism, also referred to as “trapping” or “uptake,” that enables thyroid concentration of iodide far in excess of that in the plasma [3, 4]. A plasma membrane protein, the sodium/iodide symporter (NIS), is responsible for iodide transport. Symporter activity is influenced primarily by pituitary thyrotropin, also called thyroid-stimulating hormone (TSH), which increases the transport of iodide. The trapped iodide subsequently undergoes organification and incorporation into thyroid hormones.
Iodide is accumulated, though not organified, in other organs including the salivary glands, stomach, mucous glands, skin, breast, and placenta, which may be associated with undesirable consequences for the clinical use of radioiodine. After therapeutic administration of I-131 for thyroid cancer, uptake in the salivary glands and gastric mucosa may cause sialitis and gastritis, respectively, while activity in the skin and mucous secretions may increase environmental contamination and interfere with image interpretation [5–7]. Iodide uptake by the placenta and mammary glands exposes the fetus and the nursing child to unacceptable amounts of radiation from both the therapeutic and diagnostic uses of I-131 [8, 9].
Other anions, including pertechnetate, thiocyanate, and perchlorate, also are accumulated by the thyroid gland. The uptake of pertechnetate is the basis for Tc99m-pertechnetate scintigraphy. Thiocyanate, derived from certain foods, decreases thyroid accumulation of iodine and may exacerbate iodine deficiency. Perchlorate has diagnostic and therapeutic applications discussed later.
7.2.2 Hormone Synthesis
Iodide transported via NIS at the basolateral cell membrane is converted to an oxidized form at the apical surface of the cell by thyroid peroxidase (TPO) in the presence of hydrogen peroxide. Oxidation of iodide permits its binding to the amino acid tyrosine. Synthesis of hormone takes place in thyroglobulin, a glycoprotein, which is produced in the thyroid cell and extruded into the colloid. Iodine combines with tyrosine in thyroglobulin to form monoiodotyrosine (MIT) and diiodotyrosine (DIT). Subsequently, the iodotyrosines are coupled, with the formation of thyroxine (T4) and triiodothyronine (T3). The coupling reaction also is mediated by peroxidase.
Decrease in peroxidase, associated with certain congenital and acquired thyroid disorders, impairs organic iodination and increases the proportion of unbound intrathyroidal iodine. Potassium perchlorate in pharmacological doses discharges unbound iodine from the thyroid. This is the basis for its use in the “perchlorate discharge test” to detect an organification defect [10–12] and in the treatment of thyroid dysfunction caused by amiodarone, an iodine-rich drug (see later).
7.2.3 Release of Hormone and Thyroglobulin
In response to TSH, a small amount of colloid is engulfed by the epithelial cell and proteolyzed, with release of T3 and T4, which diffuse into the circulation. Thyroglobulin not undergoing proteolysis also enters the circulation in small quantities. The serum thyroglobulin has been used as a tumor marker in differentiated thyroid cancer. Thyroglobulin decreases and eventually becomes undetectable following thyroidectomy and I-131 ablation, and its subsequent rise indicates a recurrence. TSH stimulation, by promoting colloid endocytosis, increases the amount of thyroglobulin released. Consequently, the serum thyroglobulin is a more reliable tumor marker at high TSH levels [13, 14].
7.2.4 T3 and T4
Most of the circulating thyroid hormones are bound to plasma proteins, the free fraction comprising about 0.05 % of T4 and 0.2 % of T3. Only the free hormone has metabolic effects, and it is a more accurate measure of thyroid function than the total hormone, which varies with plasma proteins levels.
T3 is considered the active hormone. About 20–30 % of the circulating T3 is secreted by the thyroid gland, and the remainder is produced by monodeiodination of T4 in extrathyroid tissues, notably the liver, kidney, brain, and pituitary [14]. Decrease in the peripheral conversion of T4 to T3 is a basis for the use of some antithyroid drugs (see below).
Synthetic forms of thyroid hormones are commonly used for replacement and/or suppressive therapy. Thyroxine is preferred for this purpose because it has a longer biological half-life (6–7 days) compared with T3 (about 1–2 days). However, T3 has a more rapid onset of action and may be useful in selected clinical situations.
7.2.5 Antithyroid Drugs
Antithyroid drugs generally block one or more steps in the synthesis and metabolism of thyroid hormone. The thiourea derivatives (“thionamides”), including propylthiouracil (PTU) and methimazole, are the most common antithyroid agents in use [14, 15]. Both decrease hormone synthesis primarily by blocking iodine organification, while PTU alone decreases the monodeiodination of T4 to T3. These drugs also are associated with decrease in serum levels of thyrotropin receptor autoantibodies (TRAB), which are responsible for Graves’ hyperthyroidism. Methimazole or PTU may be used to control hyperthyroidism in Graves’ disease and toxic nodular goiter before I-131 treatment or thyroidectomy. In selected patients, particularly children, these drugs also are used as primary therapy for Graves’ disease. Remission occurs in a minority of patients after thionamide treatment for 1–2 years or longer. Methimazole is generally the preferred drug since PTU may be associated with serious hepatotoxicity [16].
Other drugs used for their antithyroid actions include glucocorticoids, iodides, lithium, and potassium perchlorate [14]. Glucocorticoids have a rapid inhibitory effect on the peripheral conversion of T4 to T3 and are a useful adjunct in thyroid storm. Their anti-inflammatory and cell membrane-stabilizing actions have been utilized in Graves’ ophthalmopathy and protracted subacute thyroiditis. Iodide in pharmacological amounts decreases the synthesis of thyroid hormones, permitting rapid control of hyperthyroidism in thyroid storm (see Sect. 7.5.2.1). It also blocks thyroid uptake of radioiodine and is recommended as a prophylactic measure after a nuclear reactor accident [17]. Lithium blocks the release of thyroid hormone and may be used as an adjunct for the control of severe hyperthyroidism. Potassium perchlorate decreases thyroid iodine uptake and discharges unbound iodine. It may be used for the treatment of thyroid dysfunction caused by amiodarone, a drug with a high iodine content, and after accidental exposure to radioactive iodine.
7.2.6 Summary
Synthesis and secretion of thyroid hormone are regulated primarily by thyrotropin. Circulating iodide is trapped by the thyroid epithelial cell, oxidized, and bound to tyrosine. Coupling of iodotyrosines yields T3 and T4. Thyroid peroxidase promotes oxidation of iodide, a necessary step for iodination of tyrosine, as well as coupling of iodotyrosines. Thyroid hormone action is mediated by T3. About 20–30 % of the circulating T3 is secreted by the thyroid, and the remainder is derived from the peripheral monodeiodination of T4. Among the drugs with antithyroid actions, PTU and methimazole are most commonly used. Both drugs decrease hormone synthesis, while PTU alone decreases the conversion of T4 to T3.
7.3 Thyroid Handling of Radiotracers
7.3.1 Technetium-99m-Pertechnetate
Technetium-99m-pertechnetate is widely used for imaging the thyroid gland. The popularity of this radiotracer stems from its easy availability (from portable molybdenum-99 generators) and low absorbed radiation dose (short half-life of 6 h and absence of beta emissions) [18].
Technetium-99 m-pertechnetate is trapped by the thyroid, but unlike iodine, it does not undergo organification and remains in the gland for a relatively short period. Therefore, imaging is done about 20–30 min after administration of the radiotracer. Approximately 5–10 mCi (185–370 MBq) is used. The thyroid-to-background activity ratio is not as high as that with radioiodine so that Tc99m-pertechnetate is unsuitable for imaging of metastatic thyroid carcinoma, which usually functions poorly compared with normal tissue. Imaging of ectopic mediastinal thyroid tissue also may be suboptimal due to high blood and soft tissue background activity.
7.3.2 Iodine-123
Iodine-123 has ideal characteristics for imaging the thyroid gland, with a short physical half-life of 13 h, absence of beta emissions, and high uptake in thyroid tissue relative to background [18]. However, it is less readily available and more expensive than Tc99m-pertechnetate. 123I undergoes organic binding in the thyroid gland, and imaging is usually done 4–24 h after the administration of 200–400 μCi (7.4–14.8 MBq) of radiotracer. Because of its superior biodistribution characteristics, 123I is preferred over Tc99m-pertechnetate for imaging of poorly functioning and ectopic thyroid glands. 123I also may be used for whole-body imaging in differentiated thyroid cancer (see below). Approximately 2–4 mCi (74–148 MBq) of the radiotracer is used for this purpose.
7.3.3 Iodine-131
Iodine-131 may be used for the measurement of thyroid uptake, which requires only small amounts of radiotracer. It is no longer used for routine imaging of the thyroid gland because of a high absorbed radiation dose related to the long physical half-life of 8 days and beta emissions. I-131, however, continues to be valuable for the detection of metastases and recurrences in differentiated thyroid cancer [13, 18, 19]. Following appropriate patient preparation to increase TSH levels (see Sect. 7.4.3), 2–4 mCi (74–148 MBq) of I-131 is administered, and imaging is performed 48–96 h later. Radioiodine imaging has diagnostic as well as prognostic value. Iodine-avid tumors tend to have well-differentiated histological features and a favorable prognosis, whereas tumors that do not accumulate iodine are likely to be less differentiated and more aggressive [13, 20, 21].
Iodine-131 delivers a high absorbed radiation dose to the thyroid, with relative sparing of non-thyroid tissues. It is therefore ideal for the treatment of thyroid disease and used extensively in the management of Graves’ disease, toxic nodular goiter, and differentiated thyroid cancer.
7.3.4 Fluorine-18-Fluorodeoxyglucose
Positron emission tomography (PET) with 18F-fluorodeoxyglucose (FDG) is used in evaluating a variety of neoplasms including differentiated thyroid cancer. Imaging is possible for two reasons. First, malignant tumors derive energy from a higher rate of glycolysis, so that the uptake of glucose (and FDG) is increased. Second, unlike glucose, FDG is not metabolized completely and retained longer within the tumor. In differentiated thyroid cancer, FDG may be used to identify metastases not visualized at radioiodine imaging and to assess prognosis. Lesions that accumulate FDG tend to follow a more aggressive course than lesions that are not FDG-avid [22, 23]. Whole-body FDG-PET, therefore, is useful in evaluating high-risk thyroid cancer. Patient preparation is similar to that for radioiodine scintigraphy, since the uptake and diagnostic sensitivity of FDG are increased by TSH stimulation [24, 25]. Focal uptake of FDG within the thyroid gland, an occasional finding at evaluation of non-thyroid cancers, may be related to a benign or malignant pathology [26].
7.3.5 Summary
Technetium-99 m-pertechnetate is trapped but not organified by thyroid tissue. Imaging with this radiotracer is limited to the intact thyroid gland. 123I and I-131 are trapped and organified and provide higher thyroid-to-background uptake ratios. Both tracers are used to detect thyroid cancer metastases, while 123I is also used for imaging the thyroid gland. I-131 delivers a high absorbed radiation dose to thyroid tissue and is a mainstay in the management of Graves’ disease, toxic nodular goiter, and differentiated thyroid cancer. Imaging and treatment of thyroid cancer metastases with I-131 require high TSH levels. 18F-FDG, a glucose analogue, is accumulated in various malignant tumors including differentiated thyroid cancer. FDG-PET is particularly useful in high-risk thyroid cancer, where it may detect metastases not visualized at radioiodine imaging and provide prognostic information. Tumor uptake of FDG is increased by TSH stimulation.
7.4 TSH and Thyroid Function
7.4.1 TSH Secretion
Thyrotropin-releasing hormone (TRH), a tripeptide originating from the hypothalamic median eminence, stimulates the secretion and synthesis of thyroid-stimulating hormone (TSH, thyrotropin), a glycoprotein, by the anterior pituitary. TSH comprises an alpha unit, also present in other anterior pituitary hormones (FSH, LH), and a beta unit responsible for its specific actions. It acts on specific membrane-bound receptors of the thyroid epithelial cell, activating the adenylate cyclase system and increasing sodium/iodide symporter expression. As a result, the transport of iodide, synthesis of hormone, and release of T3, T4, and thyroglobulin are increased.
The production and release of TSH are influenced by the concentration of T3 within the pituitary. When the T3 concentration falls below a “set point,” TSH secretion increases, and synthesis and release of thyroid hormones are accelerated. Conversely, when the T3 level rises above the set point, TSH release is inhibited. In addition to its pituitary effect, T3 inhibits hypothalamic TRH release. Other mechanisms reported more recently include the inhibitory actions of the released TSH on TRH secretion and on TSH receptors in the pituitary itself. In sum, TSH secretion is influenced by thyroid-to-pituitary, thyroid-to-hypothalamus, pituitary-to-hypothalamus, and pituitary-to-pituitary feedback control mechanisms, which combine to reduce fluctuations in circulating T3 and T4 [14, 27, 28]. In the rare condition of partial tissue resistance to thyroid hormone, the pituitary fails to respond to increasing T3 levels, so that TSH continues to be secreted and serum TSH and thyroid hormones are both elevated. Individuals with this condition may become hyperthyroid if tissue resistance is limited to the pituitary or remain euthyroid if resistance is generalized [29].
In addition to regulation of thyroid function, TSH promotes thyroid growth. If thyroid hormone synthesis is chronically impaired, as in iodine deficiency and autoimmune thyroid disease, chronic TSH stimulation eventually may lead to the development of a goiter.
7.4.2 Serum TSH in Thyroid Disorders
The serum TSH is a sensitive and specific marker of thyroid function. Normal serum TSH is about 0.45–4.5 μunits/ml, and levels up to 20 μunits/ml are considered normal in newborns because of the contribution of maternal TSH. During early gestation, TSH tends to be at low normal (at times below normal) levels, which coincide with a surge in human chorionic gonadotropin (hCG) release. Serum TSH is increased in primary hypothyroidism and decreased in hyperthyroxinemia of all etiologies except for the uncommon entity of thyrotropin-induced hyperthyroidism.
The availability of high-sensitivity assays, which can accurately measure very low TSH levels, has significantly improved the ability to diagnose mild hyperthyroidism. Currently, most assays can detect levels to 0.01 μunits/ml or lower and are particularly helpful in establishing subclinical hyperthyroidism in nodular thyroid disease and athyrotic persons receiving replacement levothyroxine therapy [27, 30]. Subclinical hyperthyroidism in older individuals may be associated with adverse effects on the heart and bone mineral density [31–35].
The serum TSH is also a sensitive marker of hypothyroidism. As such it is commonly used to detect hypothyroidism in Hashimoto’s disease, newborns, and hyperthyroid patients treated with I-131. The TRH stimulation test measures the TSH response to TRH. It was used in the past for the diagnosis of subtle thyroid dysfunction including central hypothyroidism but has been largely abandoned with the emergence of high-sensitivity TSH assays [36].
7.4.3 Manipulation of TSH Levels
7.4.3.1 Suppressing TSH Levels
The secretion of TSH is suppressed with exogenous thyroid hormone to avoid stimulation of tumor growth in patients with differentiated thyroid cancer and to decrease thyroid size or arrest thyroid growth in the early stages of goiter development. While levothyroxine (T4) is the traditional thyroid hormone preparation for this purpose, regimens combining T4 and T3 are currently under investigation. Not infrequently, patients receiving levothyroxine are referred for a nuclear uptake and scan, requiring hormone withdrawal to allow the recovery of the hypothalamus-pituitary-thyroid axis. It may take as long as 8 weeks for recovery and for return of radioiodine uptakes to baseline values; however, shorter periods of up to 3 weeks may suffice for evaluating nodular function.
7.4.3.2 Increasing TSH Levels
Stimulation with TSH increases thyroid function and thyroid uptake of radioiodine. This principle is used in differentiated thyroid cancer for the detection and treatment of thyroid remnants and thyroid cancer metastases with radioiodine [13, 37]. Thyroid-stimulating hormone levels are allowed to rise to 30–50 μunits/ml or higher after withholding thyroid hormone supplements or after administering recombinant human TSH. The latter is gaining in popularity since it shortens the preparation time and avoids a period of hypothyroidism [37–41]. In thyroid cancer, recombinant TSH is approved for routine use in diagnosis, i.e., for scintigraphy and monitoring of serum thyroglobulin, and for radioiodine ablation of thyroid remnants after thyroidectomy. As noted earlier, PET with fluorodeoxyglucose is optimal at high TSH levels, and it may be combined with radioiodine imaging and thyroglobulin measurement in selected patients [22–25].
Recombinant human TSH may have the potential to facilitate the treatment of large nodular goiters with I-131. Radioiodine uptake in these goiters is usually low and heterogeneous. As a result, large and multiple therapeutic I-131 doses may be needed to reduce goiter volume and cure the associated hyperthyroidism. In a number of studies, a small dose of recombinant TSH resulted in a more uniform I-131 distribution, a higher 24-h uptake, and an increased therapeutic efficacy [42–44].
7.4.4 Summary
Thyroid-stimulating hormone (thyrotropin) promotes iodide transport and the synthesis and release of thyroid hormone and thyroglobulin. The secretion of TSH is modulated by the hypothalamus-pituitary-thyroid axis. The serum TSH level is a sensitive and specific marker of primary hyperthyroidism and hypothyroidism and is particularly valuable for diagnosing subclinical thyroid dysfunction. The suppression of TSH secretion with exogenous thyroid hormone may help reduce goiter size and limit the growth of thyroid cancer. In athyrotic patients with differentiated thyroid cancer, a high serum TSH is needed for radioiodine/FDG imaging, thyroglobulin measurement, and I-131 treatment. The serum TSH may be increased by withdrawing thyroid hormone or by administering recombinant human TSH.
7.5 Iodine Intake and Thyroid Function
7.5.1 Iodine Deficiency
The daily requirement for iodine is about 150 μg, increasing to roughly 200–250 μg during pregnancy. Iodine deficiency is most prevalent in the mountainous regions of the Himalayas, Alps, and Andes and in some lowlands remote from the ocean. Iodine deficiency alone or in combination with goitrogens present in certain foods results in decreased thyroid hormone synthesis [45, 46]. Selenium deficiency may be a contributing factor. Reduced synthesis of thyroid hormone is compensated, at least in part, by increased TSH secretion, resulting eventually in goiter formation. Because an adequate supply of thyroid hormone is needed for fetal neurological development, maternal and fetal hypothyroidism resulting from iodine deficiency is associated with varying degrees of neuropsychological deficits including cretinism [47–49].
7.5.2 Iodine Excess
7.5.2.1 Thyroid Autoregulation
Thyroid hormone homeostasis is maintained by an intrathyroid autoregulatory mechanism in addition to the hypothalamus-pituitary-thyroid axis. When intrathyroid iodine concentrations are significantly increased, the rate of thyroid hormone synthesis is decreased, with a reduction in iodothyronine synthesis and decrease in the DIT/MIT ratio. This response is referred to as the Wolff-Chaikoff effect [50].
The amount of intrathyroid iodine needed to trigger the Wolff-Chaikoff effect varies, depending on prior long-term iodine intake and thyroid function. Barring other mechanisms, continued exposure to large amounts of iodine would eventually lead to hypothyroidism, with compensatory increase in TSH and development of goiter. While this does occur occasionally (see below), adaptation or “escape” from the effects of chronic iodide excess is more likely. Adaptation appears to be the result of an absolute decrease in iodide transport, so that intrathyroid iodine is reduced to levels that allow resumption of hormone synthesis.
The inhibitory effect of iodides on thyroid function is utilized clinically for prompt control of severe hyperthyroidism and thyroid storm. In Graves’ disease, large doses of iodide decrease not only hormone synthesis but also hormone release [51, 52]. Since escape from the inhibitory effect is likely, iodide therapy is only a short-term measure for lowering thyroid hormone levels rapidly.
7.5.2.2 Thyroid Dysfunction
Iodine excess may lead to hyperthyroidism or hypothyroidism [50, 53, 54]. Iodine-induced hyperthyroidism, referred to as jodbasedow, characteristically occurs in persons with hyperplastic thyroid glands. Hyperthyroidism occurring after iodine supplementation in endemic goiter areas is a classical example. Iodine-containing medical products, including amiodarone, radiographic dyes, and kelp, also have the potential to cause jodbasedow [55–58]. Amiodarone, a cardiac antiarrhythmic drug, is perhaps the commonest source of iodine today. Each 200 mg tablet yields about 7 mg free iodine, while the daily requirement is only 0.15 mg. Amiodarone-related hyperthyroidism may be related to another mechanism. The drug may cause thyroiditis, which is discussed later (see Sect. 7.7).
Hypothyroidism related to increased iodine intake results from the inability to escape from the Wolff-Chaikoff effect. It is more frequent in iodine-sufficient areas, where autoimmune disease is more common than nodular disease [53–55]. In the past, “iodide goiter” with or without hypothyroidism was related to the use of iodine solutions as mucolytic agents in bronchial asthma, often with reversal of clinical manifestations after stopping the drug. A similar condition has been reported from ingestion of large quantities of (iodine-rich) seaweed in the coastal regions of Japan [59].
7.5.2.3 Iodine and Autoimmune Thyroid Disease
Iodine appears to have another, more insidious effect on the thyroid. In regions that were previously iodine-deficient, a rise in autoimmune thyroid disease has been observed after the institution of iodine supplementation in foods [54, 60]. Experimental work in animals confirms an association between iodine and autoimmunity, probably related in part to the greater antigenic potential of highly iodinated thyroglobulin [61]. Autoimmune thyroid disease and associated disorders are discussed under “Hashimoto’s Disease.”
7.5.3 Summary
Excessive amounts of iodine may cause hypothyroidism or hyperthyroidism. A significant increase in thyroid concentration of iodine may initiate an autoregulatory response, the Wolff-Chaikoff effect, which decreases hormone synthesis. Although this effect is usually temporary, occasionally it may be sustained and lead to hypothyroidism. Iodine-induced hypothyroidism is more frequent in iodine-replete regions with a high prevalence of autoimmune thyroid disease. Excessive iodine also may lead to hyperthyroidism. This may occur in individuals with nodular thyroid glands, and it is more common in iodine-deficient areas. In addition to its effects on thyroid function, iodine is believed to promote the development of autoimmune thyroid disease, a view supported by epidemiological and experimental evidence.
7.6 Endemic Goiter
Endemic goiter is attributed primarily to iodine deficiency, possibly in association with selenium deficiency or goitrogens. Goitrogens are present in certain foods and chemicals and cause either decreased synthesis or increased metabolism of thyroid hormone.
7.6.1 Goitrogens
Certain foods including cassava and bamboo shoots contain cyanogenic compounds, which may interfere with thyroid accumulation of iodine and exacerbate iodine deficiency [62]. Other foods with goitrogenic potential include pearl millet and plants from the brassica family [63].
Various chemicals may alter thyroid hormone metabolism and lead to the development of goiter. Contamination of drinking water with ammonium perchlorate from discarded rocket fuel was a concern. However, the suggested regulatory limit for perchlorate concentration in water is well below the amount needed to block iodine uptake [64]. Cigarette smoking has been linked to thyroid disease and aggravation of Graves’ ophthalmopathy. The effects presumably are mediated in part by thiocyanate [65]. Other industrial chemicals and drugs may induce hepatic enzymes that accelerate the metabolic elimination of thyroid hormone [66, 67].
7.6.2 Pathophysiology
The thyroid enlarges primarily in response to TSH stimulation resulting from inefficient hormone synthesis. There is natural heterogeneity in cellular growth and response to TSH, and rapid proliferation of thyrocytes with a growth advantage leads eventually to the development of nodules. An additional mechanism for nodule formation involves the activation of the adenylate cyclase system, usually by somatic mutations of the TSH receptor, with increase in cell replication rates [68–71]. Evidence of such mutations has been found in both solitary nodules and nodules associated with multinodular goiters. The development of toxic nodular goiter occurs over a period of years, if not decades, with gradual transition of cell clones to micronodules and subsequently to macronodules of sufficient size to cause hyperthyroidism. The disorder, therefore, is typically seen in older individuals.
Hyperthyroidism associated with nodular goiter is often subclinical, with a suppressed TSH and a normal free T4. Nonetheless, treatment with I-131 or surgery is generally recommended in the elderly because of increased risk of osteopenia and of adverse cardiovascular sequelae including atrial fibrillation [31–35]. Suppressive levothyroxine therapy is often attempted to arrest nodular growth in euthyroid patients, but is rarely successful since the nodules are largely independent of TSH control [72].
Hyperfunctioning nodules may become “cold” or nonfunctional due to hemorrhage and necrosis. Cold nodules also may be caused by the failure of iodide transport with aging, rapid proliferation of cells with decreased function, and malignant transformation.
7.6.3 Radionuclide Procedures
Toxic multinodular goiters typically show irregular distribution of radioiodine or technetium-pertechnetate and a normal or mildly elevated 24-h radioiodine uptake. The irregular tracer distribution is consistent with heterogeneity in cell function and growth and the presence of micro- and macronodules (Fig. 7.2). Large and discrete hyperfunctioning nodules may be associated with poor uptake in the extranodular thyroid tissue. The latter consists of “suppressed” normal tissue and/or small autonomous nodules with relatively less tracer accumulation. Following I-131 treatment, the areas that were previously “cold” may appear more active. A dominant nonfunctioning nodule may be related to a number of causes but may require additional diagnostic work-up to exclude malignancy.
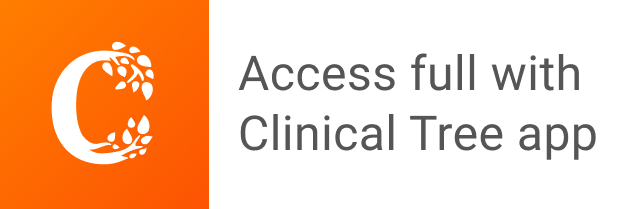