, Leonid Tiutin2 and Thomas Schwarz3
(1)
Russian Research Center for Radiology and Surgery, St. Petersburg, Russia
(2)
Department of Radiology and Nuclear Medicine, Russian Research Center for Radiology, St. Petersburg, Russia
(3)
Department of Nuclear Medicine Division of Radiology, Medical University Graz, Graz, Austria
Abstract
Central nervous system (CNS) tumors account for 4–5% of all oncological diseases and occur equally frequently in men and in women. These neoplasms are the third most common cause of death in the adult population. In Russia, every year four to seven cases of primary brain tumors are reported per 100,000 of the population, out of which 70% of patients die, and severe disability is observed among survivors in 30–40% of cases. In spite of improving surgical and conservative treatment methods, a reduction in the number of primary tumors is still not observed and the frequency of metastatic brain lesion cases is growing. So, over the last three decades the number of primary tumor cases has grown on average by 1.5 times, while the frequency of metastatic lesions has increased sixfold (Roelcke and Leenders 2001). Such an abrupt increase in frequency of metastatic brain lesions is due to their growing detectability owing to medical visualization methods; it is also connected with the tendency of growing survival rate among patients with extra-brain tumor localization, which simultaneously leads to more frequent brain metastases.
19.1 Clinical-Morphological Characteristics of Focal Brain Lesions
Central nervous system (CNS) tumors account for 4–5% of all oncological diseases and occur equally frequently in men and in women. These neoplasms are the third most common cause of death in the adult population. In Russia, every year four to seven cases of primary brain tumors are reported per 100,000 of the population, out of which 70% of patients die, and severe disability is observed among survivors in 30–40% of cases. In spite of improving surgical and conservative treatment methods, a reduction in the number of primary tumors is still not observed and the frequency of metastatic brain lesion cases is growing. So, over the last three decades the number of primary tumor cases has grown on average by 1.5 times, while the frequency of metastatic lesions has increased sixfold (Roelcke and Leenders 2001). Such an abrupt increase in frequency of metastatic brain lesions is due to their growing detectability owing to medical visualization methods; it is also connected with the tendency of growing survival rate among patients with extra-brain tumor localization, which simultaneously leads to more frequent brain metastases.
Classification of brain tumors is based on the topographic-anatomic and pathologomorphological principles. The former determines the localization of the neoplasm and is important to understand the clinical picture, including the character of neurologic lesion. According to this principle, tumors are divided into supratentorial and subtentorial types and they are also distinguished depending on lateralization and relations to intra-cerebral structures. The latter principle reflects the degree of tumor differentiation, tumor growth characteristics, its tendency to malignization and metastases; it is necessary in making the prognosis of the disease and in choosing adequate treating. There are a lot of classifications of brain and spinal tumor, but the International Classification of Tumors of the CNS adopted in Lyon in 2000 has become the most widespread.
19.2 Histological Classification of Tumors of the CNS
1.
Tumors out of neuroepithelial tissues
1.1.
Astrocytic tumors
1.1.1.
Astrocytoma: fibrillary, protoplasmatic, large-cell astrocytoma
1.1.2.
Anaplastic (malignant) astrocytoma
1.1.3.
Glioblastoma: giant-cell glioblastoma,
1.1.3.1.
Gliosarcoma
1.1.4.
Pilocytic astrocytoma
1.1.5.
Pleomorphic xanthoastrocytoma
1.1.6.
Subependymal giant-cell astrocytoma (tuberous sclerosis)
1.2.
Oligodendroglial tumors
1.2.1.
Oligodendroglioma
1.2.2.
Anaplastic (malignant) oligodendroglioma
1.3.
Ependymal tumors
1.3.1.
Ependimoma (papillary and clear cell ependimoma)
1.3.2.
Anaplastic (malignant) ependimoma
1.3.3.
Mixopapillary ependimoma
1.3.4.
Subependimoma
1.4.
Mixed gliomas
1.4.1.
Oligoastrocytoma
1.4.2.
Anaplastic (malignant) oligoastrocytoma
1.4.3.
Others
1.5.
Vascular plexus tumors
1.5.1.
Vascular plexus papilloma
1.5.2.
Vascular plexus carcinoma
1.6.
Gliomatosis cerebri
1.7.
Neuronal and mixed neuron-glial tumors
1.7.1.
Gangliocytoma
1.7.2.
Ganglioglioma
1.7.3.
Anaplastic (malignant) ganglioma
1.7.4.
Disembryoplastic neuroepithelial tumor
1.7.5.
Central neurocytoma
1.7.6.
Paraganglioma
1.7.7.
Olfactory neuroblastoma (esthesioneuroblastoma)
1.7.7.1.
Olfactory neuroepithelioma
1.8.
Parenchymatous tumors of the pineal body
1.8.1.
Pineocytoma
1.8.2.
Pineoblastoma
1.9.
Embryonal tumors
1.9.1.
Medulloepithelioma
1.9.2.
Neuroblastoma
1.9.3.
Ependymoblastoma
1.9.4.
Primitive neuroectodermal tumors
1.9.4.1.
Medulloblastoma: desmoplastic medulloblastoma, medullomyoblastoma, melanin-containing medulloblastoma
2.
Tumors of cranial and spinal nerves
2.1.
Neurinoma
2.2.
Neurofibroma
2.3.
Malignant neurinoma
3.
Tumors of meninges
3.1.
Tumors of meningothelial cells
3.1.1.
Meningoma: meningothelial, fibroblastic, transitional (mixed), psammomatous, angiomatous, secretory, clear cell meningomas
3.1.2.
Atypical meningoma
3.1.3.
Anaplastic (malignant) meningoma
3.2.
Mesenchymal, non-meningothelial tumors
Benign:
3.2.1.
Osteochondral tumors
3.2.2.
Lipoma
3.2.3.
Fibrous histiocytoma
Malignant:
3.2.5.
Hemangiopericytoma
3.2.6.
Chondrosarcoma
3.2.7.
Malignant fibrous histiocytoma
3.2.8.
Rabdomyosarcoma
3.2.9.
Meningeal sarcomatosis
3.3.
Primary melanocytic lesions
3.3.1.
Malignant melanoma
3.4.
Tumors of unclear origin
3.4.1.
Hemangioblastoma
4.
Lymphomas and tumors of blood-making tissue
4.1.
Malignant lymphoma
4.2.
Plasmocytoma
5.
Tumors of germ cells
5.1.
Germinoma
5.2.
Embryonal carcinoma
5.3.
Endodermal sinus (yolk sac) tumor
5.4.
Chorioncarcinoma
5.5.
Teratoma
6.
Cysts and tumor-like lesions
6.1.
Rathke’s pouch cyst
6.2.
Epidermoid cyst
6.3.
Dermoid cyst
6.4.
Colloid cyst of the III ventricle
6.5.
Entherogenic cyst
6.6.
Neuroglial cyst
6.7.
Granular cell tumor (choristoma, pituicytoma)
6.8.
Neuronal hamartoma of hypothalamus
7.
Tumors of the region of the Turkish saddle
7.1.
Hypophyseal adenoma
7.2.
Hypophysis cancer
7.3.
Craniofaringioma
8.
Tumors ingrown into cranial cavity
8.1.
Paranglioma (chemodectoma)
8.2.
Chordoma
8.3.
Chondroma
8.4.
Chondrosarcoma
8.5.
Cancer
9.
Metastatic tumors
10.
Unclassifiable tumors
Gliomas and meningomas make up more than 65–70% of all primary brain neoplasms, another 15–20% are neurinomas and hypophyseal adenomas, and only some 10–15% fall in the remaining large group of tumors (Ribom et al. 2001). Among malignant neoplasms, gliomas (77%) and metastases from extra-brain tumors prevail.
Prognosis in anaplastic astrocytomas (AA) and glioblastomas (GB) is unfavorable. In spite of active tactics of neurosurgeons and radiologists relative to these tumors, the life span of GB patients does not exceed 2–3 years (Baba et al. 1997; Castillo 1998). The development of the tumor process depends on the degree of malignancy (aggressiveness), growth rate and tumor localization. For example, in glioblastoma gross decompensation of the tumor process may be observed within several months and even days, while omitting other phases. At the same time, slow-growing benign meningiomas and astrocytomas may remain for years in the phase of clinical compensation or subcompensation.
The dissemination of malignant gliomas is possible by way of invasion or metastasizing. These tumors are able to extend to adjacent brain lobes and even to the hemispheres. At the same time, meningiomas are usually well delimited from the medullary substance and are characterized by slow expansion growth. Changes in brain tissues are due to the expansion of the neoplasm with consequent compression of tissue, as well as to the damaging effect of toxic factors related to the vital activity of tumor cells, and resulting in the development of oedema and necrosis of adjacent brain tissues (Martinez-Mata et al. 1999; Otte 1999). Among the earliest clinical manifestations of brain tumors, convulsive seizures, paresthesias, headaches, (rarer) central vomiting, vertigos, pareses and paralyses prevail.
Tumor process in the skull base may be accompanied by atrophic changes of optic nerves, and appearance of congested papilla in the eye ground.
Tumors of the glial family have in common their origin from astrocytic tissue, presence of more or less apparent glial-fibrous component, the infiltrative character of growth and tendency to metastases and relapses. It should be noted that in the absence of treatment more than 10% of benign astrocytomas malignize. At the same time, after non-radical surgical intervention or conservative treatment more than 50% of neoplasms transform (Piepmeier 1987).
Meningiomas grow out of meninx cells and usually adjoin bone structures of the skull (Black 1993), quite often infiltrating them (Ringel et al. 2007). Meningiomas most often localize in the region of the parietal, frontal and temporal bones of the skull, parasagittally, in the region of the falx cerebris and sinuses (falx meningiomas). Basal meningiomas most often proceed from the wings of the sphenoid bone, tubercle of the Turkish saddle, olfactory fossa and parasellar structures. A distinction of basal meningioma is lesion cerebral nerves and the vessels of the brain base. It is due to the involvement in the tumor process of adjacent structures, nerves and vessels (Friedlander et al. 1999). Meningiomas of the posterior skull fossa more often grow from petrosal bones. In these cases they have to be differentiated from neurinomas of acoustic nerve and cholesteatomas (Krayevskiy et al. 1982). The structure of meningiomas may be lobulous or solid. Benign meningiomas more often have a homogenous structure, while malignant ones are characterized by the presence of calcification, hemorrhages, necroses, cysts (Yamada et al. 1996). In malignant meningiomas, peritumorous oedema may be also present. They are distinguished by their expansive growth and apparent mass effect (Stafford et al. 1998; Mantle et al. 1999).
The differentiation of tumors by degree of malignancy is crucial both for disease prognosis and for the choice of therapeutical management. For example, with tumors of degrees III–IV life span is in most cases only 9–10 months. With tumors of degrees I-II, the 5-year survival rate reaches 50–75% (Kleihues et al. 1993; Groos et al. 1998).
It should be noted that the clinical signs of tumors are not pathognomonic and can be observed in many diseases. The clinical manifestations of tumors in most cases are related to their localization and effect on adjacent brain structures, which often enables determination of their location; however, the moment of the appearance of pathological symptoms and their intensity may range within quite wide limits. The diversity of pathologic processes underlying CNS masses as well as the similarity of neurologic symptoms restrains the possibilities of their clinical differential diagnosis.
19.3 Methods of Radiodiagnosis of Brain Tumors
Under contemporary conditions the diagnosis of volumetric brain formations is provided by a complex examination consisting of clinical-biological and instrumental methods (Konovalov et al. 1997). During such an examination, the following main tasks are to be solved: the identification of the pathologic process (nosologic diagnosis), spatial localization (topical diagnosis) of the formation, determination of its biological nature (histological diagnosis), detection of continued growth, assessment of response to treatment. Possible choice of a parcel of the neoplasm for puncture biopsy, monitoring of the state of benign tumors at high risk of malignization also have some significance.
In solving these problems, high-tech radiodiagnostic methods (MRI, CT, SPECT and PET) are of paramount importance (Konovalov et al. 1997; Brunelle 2000; Kornienko and Pronin 2006).
X-ray (craniography) for a long time was the only method of radiodiagnosis of brain tumor and skull lesions. However, this method permits to obtain information only on the state of bone structures and consequently to judge indirectly, on the basis of secondary changes occurring in them, the presence of brain masses. In the mid 1950s the method of roentgen angiography appeared which permitted to solve the problem of visualization of brain vessels (Konovalov et al. 1997). The method is still successfully applied in neurooncological clinical practice, primarily in X-ray endovascular interventions. However, X-ray angiography is an invasive examination method and it does not always allow the differential diagnosis of formations and assessment of their volume and influence on adjacent brain structures.
A breakthrough in radiodiagnosis is associated with the appearance and development of CT and MRI. These methods have a high resolving capacity. They enable the detection of structural changes occurring in the brain in the presence of different pathological processes and to obtain fairly accurate information on solid brain masses [information on the location, form, dimensions and structure of brain masses (Konovalov et al. 1997)].
Compared with CT, MRI has a number of advantages, among which count high contrast resolution, possible assessment of different tissue characteristics, and multiprojection image reconstruction, all of which make MRI the method of choice in neurooncology. MRI permits to assess structures situated near the base of the skull, which are not well visualized by CT due to frequent presence of artifacts. Absence of radiation exposure renders this method particularly valuable for pediatrics. CT is a relatively cheap and available method and is used primarily in urgent diagnosis in order to exclude brain formations. CT is the only method permitting the detection of calcium deposits inside the tumor, which may be important for differential diagnosis. CT is not efficient in presence of isodense formations.
CT and MRI in neurooncology have proved to be most efficient with use of contrast effect (Kornienko and Pronin 2006; Sergeev et al. 2007). Admittedly, it should be confessed that in spite of much progress in diagnosis and differential diagnosis of brain tumors due to the introduction of CT and MRI into clinical practice, many functional matters are still outside the range of these methods of diagnosis. This concerns first of all the biological characteristics of tumors, such as the state of metabolism, degree of malignancy of tumor cells, their reparative capacities, vascularization, DNA synthesis rate, sensitivity to hypoxia, etc. Using contrast in MRI helps to determine precisely the structure, volume and localization of formations, and their relations to surrounding tissues, as well as the state of the blood–brain barrier. However, the experience of using contrast MRI has shown that the level of accumulation of contrast agent depends on the state of microcirculation, degree of change in permeability of the blood–brain barrier, volume of intercellular space in the formation under evaluation and that it does not always permit assessment of the nature of lesion (Sergeev et al. 2007). Differentiating radiation necrosis from continued growth of tumors is not possible. For example, presence of changed or damaged tissues in the post-operation region under examination by means of contrast MRI may give rise to false-positive diagnosis of tumors due to injuries to the blood–brain barrier occurring in this case (Hagge et al. 2001). The specificity of MRI with contrast enhancement in the diagnosis of glioma relapses does not exceed 70% (Yamada et al. 1999).
CT or MRI evaluation of the response of neoplasms to treatment is based, as a rule, on detecting changes in dimensions and structure of formations. However, these changes, in case of successful treatment, are reflected in scans only after a several-month lapse. Therefore, the early-term evaluation of treatment efficiency is difficult. Besides, a change in dimensions of pathologic formations or in their anatomic structure cannot always serve as a reliable criterion for the biological state of tumor cells and for the efficiency of treatment. The most objective information for these purposes can be obtained by means of methods of radionuclide diagnosis.
Methods of radionuclide visualization were described by G. Moor in 1948 and started to be used in differential diagnosis of volumetric brain formations in late 1960s. Using radionuclide diagnosis in neurooncology is based on the possibility of visualizing malignant brain neoplasms with some radiopharmaceuticals (RPs). Nowadays technologies of nuclear medicine (SPECT and PET) have received the widest use among all methods of radionuclide diagnosis (Steinling 1997, Hustinx and Alavi 1999, Schwarz and Kuwert 2000).
Single photon emission computer tomography (SPECT) was developed in late sixties. The method is still developing systematically and is currently widely used in clinical practice. It should be noted that progress in the development of radionuclide diagnostic methods is associated not only with developing and improving radiodiagnostic apparatuses but also with the appearance of new diagnostic media and RPs. The following RPs are most widely used when using SPECT in neurooncology: 201TICI, 99mTc-technetryl (99mTc-MIBI), 99mTc-pyrphotech-erythrocytes, 1–3-123I-methyltyrosine (Plotkin et al. 2005) and others. These RPs are non-specific tumor markers. They are actively accumulated in malignant neoplasms and so permit their visualization. However, simultaneous uptake of these RPs in aponeurosis, lymphoid tissue and in the hypervascular regions of the head and neck is observed (Bader et al. 1998, 1999). This decreases their diagnostic value and makes them poorly informative in the differential diagnosis of patients with brain tumors. The amino acid tyrosine 1–3-123I-methyltyrosine (123I-IMT) is a tumor marker; the mechanism of its accumulation in brain tumors is identical with that of methionine uptake (Langen et al. 2006). 123I-IMT SPECT is used for determining tumor dimensions and for diagnosis of relapses (Plotkin et al. 2004, 2005). 111In-Octreotide, a marker of somatostatin receptors, is used for diagnosis of meningioma relapses. At the same time, the capacities of neoplasm diagnosis with help of these RPs are limited due to a relatively low resolving capacity of SPECT, which makes particularly difficult their use for radiotherapy planning and for planning of neurosurgical operations.
Further progress in diagnosis, and particularly in the differential diagnosis of volumetric brain formations, is associated with the development and introduction into clinical practice of PET in the late 1970s. PET differs from SPECT in its higher resolving capacity and sensitivity, possible use of attenuation correction, and possible obtention of tomographic images in the dynamic regime. As RPs for PET, biologically active substances labeled with cyclotron-generated ultra-short-lived radionuclides (USLRs) are used. 18F–fluorodeoxyglucose (18F–FDG) has become the first RP for PET. Early research has shown that uptake of 18F–FDG correlates to the degree of malignancy of brain tumors and has a certain prognostic value (Hagge et al. 2001). It has been found out that 18F–FDG PET makes it possible to assess the degree of malignancy of tumors (Herholz et al. 1993; De Witte et al. 2000) and to choose optimum sites for biopsy (Levivier et al. 1995). The method is actively used for differentiating brain lymphomas and toxoplasmosis foci in patients with immune deficiency syndrome since FDG is not accumulated in lesion foci in toxoplasmosis (Villringer et al. 1995). It has been suggested using 18F–FDG for detecting malignization of benign tumors (De Witte et al. 1996) and for differential diagnosis of the continued growth of malignant gliomas and radiation necrosis (Glantz et al. 1991). Presently, these early suggestions are being put in doubt (Wong et al. 2002; Spence et al. 2003). For example, due to high uneven glucose accumulation in the cortex, it is difficult to determine the boundaries of the tumor and in many cases the tumor itself. Besides that, benign astrocytomas are not visualized in 18F–FDG PET because of low metabolism in them. For the same reason, 18F–FDG PET has low sensitivity in detecting brain metastases (Larcos and Maisey 1996).
Compared with 18F–FDG, labeled amino acids such as 11C-methionine (11C-MET) (Benard et al. 2003; Ogawa et al. 1996) and 18F-fluoroethyltyrosine (18F-FET) (Wester et al. 1999) have a number of advantages in diagnosing brain tumors, especially benign astrocytomas and brain metastases. Owing to low accumulation of amino acids in brain tissue, these RPs permit to differentiate tumors from non-tumor diseases (Floeth et al. 2006), to precisely define tumor boundaries, its continued growth and relapses (Popperl et al. 2004; Pauleit et al. 2005), and to determine a representative part of the tumor for biopsy planning (Wieder et al. 2003; Sadeghi et al. 2007; Stockhammer et al. 2008). Besides 18F–FDG and labeled amino acids, other RPs are used for diagnosis of brain tumors. For example, PET with the marker of somatostatin receptors 68Ga-DOTA-D-Phe1-Tyr3-octreotide (68Ga-DOTATOC) is successfully used in diagnosis of meningiomas (Henze et al. 2001). PET with 18F-choline, the indicator of membrane-forming speed, permits to visualize many brain tumors and can be used for detecting brain metastases of prostate cancer. It has been suggested using 15O-water for assessing the degree of vascularization of brain tumor formations (Medvedev et al. 1996; Skvortsova et al. 2001a; Lüdemann et al. 2008). The hypoxia marker 18F-FMISO is of interest for planning and efficiency assessment of brain tumor radiotherapy (Cher et al. 2006). At the same time, using PET with the DNA synthesis marker 18F-fluorothimidine (18F-FLT), suggested for brain tumor diagnosis, proves to be inefficient due to apparent dependence of 18F-FLT uptake on the impairment of the blood-brain barrier (Saga et al. 2006).
It should be noted that high cost of automated modules and RP production on the whole imposes some constrains on their choice and production, and compels to choose the most informative of them. Of some importance is the possibility of quantitative evaluation of PET data with the help of multicompartment mathematical models developed for many RPs (Roelcke and Leenders 2001).
The improvement of the software for retrospective fusion of different modality images has marked an important stage of development and introduction of PET (Pietrzyk et al. 1994). It is known that localization of pathologic finds is often made difficult by absence of articulate anatomic reference points in the PET scans. That is why the fusion of PET and MRI or CT data is an important precondition for their correct topographic-anatomic interpretation (Paulus and Peiffer 1989). Recently an apparatus combining the capabilities of PET and CT, the so-called PET-CT, has been created (Beyer et al. 2001; Beyer and Townsend 2002). It enables to mark out the boundaries of formations and to assess their biological properties which are important for primary diagnosis of tumors and for diagnostic decision making. It should be noted that development of methods of fusing multimodal images such as PET-CT has accelerated the integration of PET into the process of radiotherapy and stereotactic biopsy planning. However, in neurooncology PET-CT does not have as significant advantages over PET alone as it does in diagnosing extra-cranial tumors, because retrospective fusion of MRI and PET scans is possible. PET-MRI, providing a combination of the advantages of PET and MRI in a single examination, seems to be more promising for the diagnosis of brain diseases. Prototypes of such scanners are being clinically tested (Seemann 2005).
On the basis of the world scientific literature, we can state that PET is universally admitted to be an important diagnostic tool in neurooncology as a promising functional method of radiodiagnosis and differential diagnosis of tumors. This is due to the technical capabilities of PET and the properties of RPs.
19.4 PET RPs Used in Neurooncology
19.4.1 18F-FDG PET
In 1982, Di Charo and coworkers first pointed out the possibility of using 18F-FDG PET for visualizing malignant brain tumors. High glucose uptake in malignant tumors is conditioned by high expression of the transporter GLUT1 and by hyperactivity of hexokinase. It has been established that the degree of blood–brain barrier injury and of vascularization of brain tumors do not have an impact on 18F-FDG uptake (Di Chiro 1987; Padma et al. 2003). Subsequently there appeared reports of using 18F-FDG for the differential diagnosis of neoplasms, detection of continued growth, treatment efficiency evaluation and disease prognosis in patients with different tumors (De Witte et al. 2000).
At the same time, it should be admitted that data on the diagnostic capabilities of 18F-FDG still remain conflicting (Lacic et al. 1997). Most authors consider there to be a direct correlation between the level of 18F-FDG uptake and the degree of malignancy of the tumor; they think that high 18F-FDG uptake is characteristic for malignant tumors (De Witte et al. 2000). Other authors are more reserved in evaluating the efficiency of using 18F-FDG for differential diagnosis of malignant brain tumors; they accentuate some shortcomings of this RP, the main of which being high glucose uptake in the cortex and low 18F-FDG uptake by some malignant tumors (Meyer et al. 2000, 2001). Undoubtedly, the physiological uptake and focal asymmetry of glucose metabolism provoke some difficulty in visualizing neoplasms situated in the cortex with use of 18F-FDG. At the same time, fusion of PET and MRI images permits delimitation of the region of interest and precise assessment of the level of metabolism in it, which in part helps solve the problem.
Numerous studies have been devoted to the choice of criteria for differentiating malignant and benign brain tumors with use of 18F-FDG (Delbeke et al. 1995, Meyer et al. 2001).
The most informative among existing data assessment methods is the one using color scale (RGB or Hot Metal) combined with measuring a semi-quantitative index, the accumulation index, which reflects the ratio of radioactivity accumulated in the tumor to the unchanged cortex.
It has been established that as the threshold value of FDG T/NT ratio grows, the sensitivity of the method decreases and its specificity increases (Kostenikov et al. 2002). When the cut-off criterion for malignant tumors is an FDG T/NT ratio ≥ 0.9, the maximum possible specificity is reached, approaching 100%, and the sensitivity is also relatively high (82%). A limitation of this approach consists of the dependence of T/NT ratio for FDG on the level of glucose metabolism in the unaffected brain tissue. It is accounted for by glucose metabolism disturbance under the general influence of the tumor, which can be observed in cortex regions both ipsilateral and contralateral with regard to the tumor.
Cases have been described when a focus of epileptic activity in parts adjacent to the tumor or situated in the area of radiation necrosis was wrongly interpreted as an area of tumor malignization (Sasaki et al. 1996). Differences in glucose uptake (FDG T/NT ratio) between tumors of degrees I-II of malignancy and those of degrees III-IV are significant, but differentiation between tumors of degrees III and IV with use of 18F-FDG is not possible (De Witte et al. 2000). In some earlier reports it was demonstrated that the level of 18F-FDG uptake in the tumor is comparable in informative value with histological conclusion (De Witte et al. 1996; Derlon et al. 2000). Presently these data are being questioned. In a number of studies (De Witte et al. 2000, Padma et al. 2003) devoted to the investigation of the widest spread neoplasm group (gliomas), it has been showed that the glycolysis rate in the tumor determined according to T/NT ratio may be used as a prognostic criterion: a high level of glucose metabolism in the tumor is estimated as an unfavorable prognostic factor. However, according to research done in recent years, this rule is not universal. In particular, high accumulation of 18F-FDG in oligodendrogliomas is due to characteristic chromosome injuries conditioning high sensitivity to chemotherapy, which is a favorable prognostic factor (Walker et al. 2004; Stockhammer et al. 2007).
So it follows with evidence that 18F-FDG PET is a highly informative means of diagnosis of malignant brain tumors. However, many aspects of use of this RP in neurooncology are still not completely determined. Among limitations of 18F-FDG PET in neurooncology, we should also rate high uptake of 18F-FDG by macrophages in foci of inflammatory alterations (Jager et al. 2001). In some cases it is possible to overcome this limitation by using delayed (two-phase) scanning. The diagnosis of benign brain tumors and their differentiation from non-tumor formations are very difficult when 18F-FDG is used.
The problems of identifying brain tumors, including benign ones, and of differentiating them from non-tumor diseases can be, as a rule, efficiently solved by PET with labeled amino acids (11C-MET or 18F-FET) (Floeth et al. 2006).
19.4.2 11C-methionine (11C-MET) and Other Labeled Amino Acids
The advantage of 11C-MET over 18F-FDG is its high uptake in neoplasms in combination with low accumulation in the cortex, which provides visualization of tumors. It has been established that 11C-MET PET enables to precisely delimit tumor boundaries, to differentiate edema from neoplasms and to detect both malignant and benign tumors (Jager et al. 2001; Chung et al. 2002; Wieder et al. 2003).
Some authors point out the high informative value of 11C-MET PET in differentially diagnosing gliomas: the sensitivity of this method is 89–90% and its specificity ranges from 94% to 100% (Skvortsova et al. 2001b; Chung et al. 2002). This method is also informative for the diagnosis of brain metastases (Tsuyuguchi et al. 2003). The causes of uptake of methionine in tumors are not completely examined. It is considered that it is uptaken in high quantities due to high proliferation rate in neoplasms. It has an impact on the active RP transfer through the cell membrane of tumors (Ribom et al. 2002). Methionine is transported to a tumor cell by means of active transfer with participation of the transporters LAT-1 and LAT-2 (Jager et al. 2001).
The most important aspect of using 11C–MET is evaluating the effects of benign brain tumor treatment, which is possible with its help (Nuutinen et al. 2000; Ribom et al. 2002). It has been determined that a decrease in uptake of this RP in tumors during treatment objectively reflects the positive effect of conservative therapy (Iwai et al. 2001; Muhr et al. 2001; Gudjonsson et al. 2000).
Recently 11C–MET has been used to choose the target in stereotactic biopsy of neoplasms (Wieder et al. 2003; Sadeghi et al. 2007). In a number of studies it has been shown that the uptake of this RP in benign gliomas is significantly lower than in malignant ones (Skvortsova 2001). Nevertheless, the value of this approach for assessing the degree of malignancy of gliomas is put to doubt (Moulin-Romsée et al. 2007). One of shortcomings of 11C–MET is its high accumulation in unchanged endocrine glands, including hypophysis. High accumulation of this RP in inflammation foci (uptake by macrophages and granulocytes) and in intracerebral hematomas in blood–brain barrier injuries has been described, which makes the differential diagnosis of tumor diseases difficult and may give rise to false-positive conclusions (Hanakawa et al. 1998).
The indicated limitations of 11C-MET have stimulated the intensive development of other RPs, such as 11C-thymidine (Jager et al. 2001), labeled amino acids including 11C-tyrosine, fluoroboronophenylalanine (Imahori et al. 1998), 18F- fluoroethyl–tyrosine (18F-FET) (Weber et al. 2000) and a number of others. 18F-FET is considered to be the most promising among the above mentioned RP. Due to a longer half-life period of 18F (compared with 11C) this RP can be transported, like 18F-FDG, which, together with simple methodology of synthesis and possibility to examine up to ten patients daily, expands the possibilities of its clinical use.
Unlike 11C-MET, 18F-FET is not accumulated in macrophages and granulocytes and therefore has a higher specificity (Stöber et al. 2006). Transport to tumor cells is not connected with crossing the blood–brain barrier and is realized by means of active transfer with help of the transporter LAT-2. Accumulation of 18F-FET in tumors depends on the degree of angiogenesis and density of tumor cells (Stockhammer et al. 2008). Unlike 11C-MET, 18F-FET does not participate in protein synthesis and is a marker of amino acid transport (Langen et al. 2006).
Some authors point out the possibility of determining the degree of malignancy of gliomas by reference to 18F-FET accumulation rate in tumor. This method is based on a lower 18F-FET uptake by benign gliomas compared with malignant ones (Pöpperl et al. 2006a).
Besides labeled glucose and amino acids, the diagnostic capabilities of other labeled biologically active natural substances have been studied, such as acetate, choline, fatty acids, putrescine, deprenyl, fluoro–dihydroxyphenylalanine (DOPA). It has been showed that 11C-acetate is better accumulated in tumors then labeled aromatic (synthetic) fatty acids, for example 11C-phenylacetate and 18F-fluorophenylacetate (Jonson and Welch 2002). 11C-Choline, being part of cell membranes, also permits the visualization of malignant gliomas and to differentiate them from benign brain tumors and non-tumor formations. 11C–Putrescine and 11C–deprenyl showed high uptake in tumors (De Reuck et al. 1999). Relatively recently, 18F-DOPA has been described as a marker of brain tumors. In our studies we have shown that the labeled fatty acid 11C-sodium butyrate (11C-SB) is accumulated in high amounts in malignant and some hypervascular benign tumors. 11C-SB permits to simultaneously assess some parameters characterizing properties of neoplasms, such as vascularization, the rate of uptake and utilization of fatty acids, and the speed of metabolic processes (Kostenikov et al. 2002).
19.5 Methodological Aspects of Using PET in Brain Tumors
19.5.1 PET Scanning
The characteristics of PET emission scanning methods for some RPs widely used in neurooncology are summarized in Table 19.1
Table 19.1
The characteristics of the methods of PET scanning
RP | The measured index | Diagnostic RP dose (MBq/m2) | Factor for calculating the effective dose in adults (mSv/MBq) | Onset of scanning (min) | Scan duration (min) | Scan regime |
---|---|---|---|---|---|---|
18F-FDG | Glucose metabolism (transmembrane transport) rate | 100 | 19.0 × 10−3 | 35–40 0 | 20 55 | Static Dynamic |
11C-MET | Protein synthesis rate | 350 | 2.0 × 10−3 | 5 | 20 | Static |
0 | 20 | Dynamic | ||||
18F-FET | Amino acid transport rate | 150 | 16.5 × 10−3 | 10 | 20 | Static |
0 | 40 | Dynamic | ||||
68Ga-DOTATOC | Somatostatin receptor level | 50 | 27.4 × 10−3 | 60 | 20 | Static |
11C-sodium butyrate | Fatty acid metabolism | 350 | 3.5 × 10−3 | 0 | 30 | Dynamic |
15O-water | Regional blood flow rate | 500 | 0.93 × 10−3 | 0 | 1 | Static |
Emission scanning is preceded by 10-min transmission scanning with calibration sources of ionizing radiation (68Ge) for attenuation correction. The effective dose for 10 min is 0.07 mSv. When PET-CT scanners are used, transmission scanning is provided by CT; the effective dose in CT is from 1.2 to 3 mSv.
From 20–30 min before to 15–20 min after the introduction of 18F-FDG the patient is sound and light insulated in order to exclude the physiological uptake of the RP in the cortex. 18F-FDG is introduced in the form of a jet intravenous injection in the volume of 1.5 mL 35–40 min before the beginning of the emission static scanning into a peripheric vein of the limbs. The diagnostic dose of 18F-FDG in brain examination makes up 100 MBq/m2 per body surface (150–250 MBq/m2 for an examination). The coefficient for calculation of the effective dose when introducing 18F-FDG is 0.019 mSv/MBq. When introducing the diagnostic dose of RP, the effective dose is from 2.85 to 4.75 mSv for an examination.
Static brain scanning lasts 20 min and is done in 2D or 3D regimes with use of the matrix 128 × 128 and with image enlargement by 1.5 times. If quantitative analysis is necessary, dynamic scanning is implemented. Dynamic scanning with 18F-FDG is done under the same conditions as static scanning. It starts immediately after RP introduction and lasts 50 more minutes.
Brain scanning with 11C-MET lasts 10–20 min and is done in the same regime as that with 18F-FDG, 5 min after the jet-mediated intravenous introduction of 350 MBk/m2 of RP. As a rule, 370–950 MBq of RP is used for one PET examination. The coefficient for calculation of the effective dose when introducing 11C-MET is 0.0074 mSv/MBq. When introducing the diagnostic dose of RP its effective dose is from 2.74 to 7.0 mSv for an examination. When a PET-CT scanner with lutetium oxyorthosilicate (LSO) or gadolinium oxyorthosilicate (GSO) crystals is used, the diagnostic dose of 11C-MET is lower than the one for PET scanner and is 250–700 MBq.
A brain scan with 18F-FET may be done in the same static or dynamic regimes as that with 18F-FDG. Patients are administered a protein-free diet 12 h before the examination. A 20-min static scanning is implemented 10 min after the jet-mediated intravenous introduction of 150 MBq/m2 of 18F-FET. As a rule, 250–300 MBq of RP are used for one examination. Dynamic scanning begins immediately after RP is introduced and lasts for 40 min. The coefficient for calculating the effective dose when introducing 18F-FET is 0.0165 mSv/MBq. When introducing the diagnostic dose of the RP the effective dose is from 4.13 to 4.95 mSv for an examination. When a PET-CT scanner with LSO or GSO crystals is used the diagnostic dose of 18F-FET is lower than that for PET and amounts to 185–200 MBq. Immediately before the emission scan, a diagnostic scan with contrast enhancement is recommended.
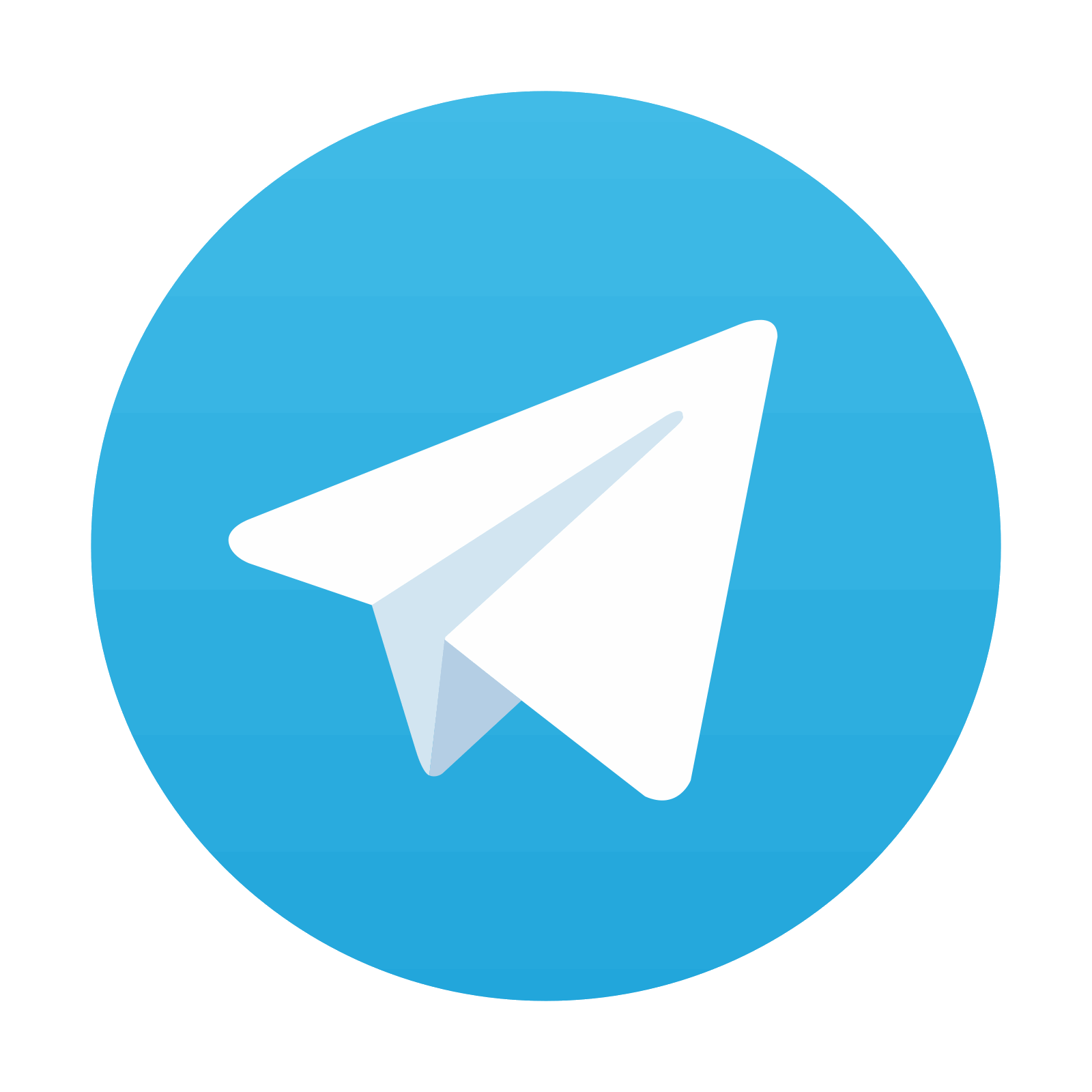
Stay updated, free articles. Join our Telegram channel
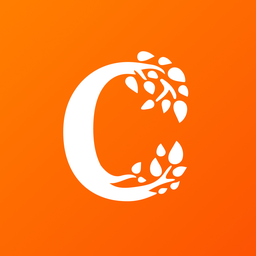
Full access? Get Clinical Tree
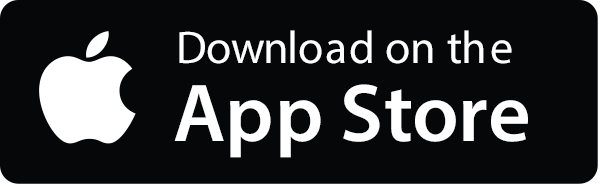
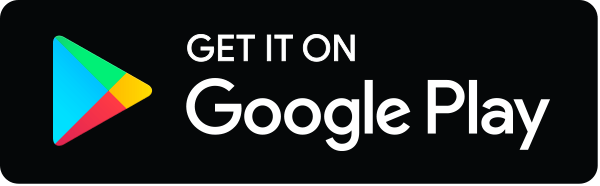