Fig. 20.1
Ultrasound cavitation
20.2.3 Ultrasound Sonochemistry
Ultrasound has been used to invent novel chemical reactions and to enhance the reaction rate. This is called sonochemistry. Ultrasonic cavitation converts acoustics into extreme physics, creating a vigorous environment. Such special physical and chemical effects provide a new route for chemical reactions that are difficult or impossible to achieve under conventional conditions. During bubble collapse, radicals can be generated due to decomposition of solvents and monomers, or rupture of polymer chains. This initiates further chemical reaction, e.g., monomer polymerization (Mason and Lorimer 1988; Kruus 1983; Price et al. 1992). Since the first report about sonochemistry by Richards and Loomis (1927), sonochemistry has initiated growing interest in many areas of synthetic chemistry, especially in polymer science.
20.2.3.1 Ultrasound Degradation
A well-known use of ultrasound is the degradation of polymers to lower molecular weights and their distribution control. Previous research suggests that ultrasonic depolymerization is a non-random process, with the chain scission occurring preferentially at the polymer chain midpoints, and with larger molecules degrading the fastest (Mason and Lorimer 1988). Another unique feature is that ultrasonic degradation reduces the molecular weight simply by splitting the most susceptible chemical bond in the chain (Gronroos et al. 2001). The factors affecting ultrasonic polymer degradation and its mechanisms have been investigated in detail, typically for polymers in solution (Price and Smith 1991; Tabata and Sohma 1980; Malhotra 1982). Moreover, by the performance of degradation in the presence of the radical scavenger, it has been proven that ultrasound can degrade polymer chains to macro-radicals (Chen et al. 1985). Reich reported that at an ultrasound intensity of 40 W and above, a significant reduction in the molecular weight of poly (lactic acid) (PLA) and poly(lactic-co-glycolic acid) (PLGA) could be observed, even after short exposure times of 30 or 20 s, respectively (Reich 1988). EI-sherif et al. (2004) also observed the degradation of PLGA under a high frequency ultrasound of 5–10 MHz. By controlling the reaction parameters influencing the degradation of polymer chains and the copolymerization of added monomers, one can produce various grafts or block copolymers with tailored structures in systems containing either a mixture of homopolymers or a mixture of polymers and monomers (Zhang et al. 1990; Fujiwara et al. 1992, 1994). Therefore, ultrasonic irradiation can provide an effective method for polymer design.
The exact mechanism of ultrasonic degradation still remains obscure, but it is well accepted that the large shear fields and instantaneous hot spots produced by ultrasonic cavitation are primarily responsible for polymer degradation (Kawasaki et al. 2007; Liu et al. 1992). It is believed that at the stage of cavity collapse induced by ultrasound waves, radiated friction forces and shock waves generate the stresses on the surface of a polymer chain, and/or more possibly within the polymer coil, resulting in macromolecular chain bond breaking in the liquid. This is similar to hydrodynamic shear degradation (Mason and Lorimer 1988). When the microbubbles collapse, polymer segments in the high-gradient shear field, near the collapsing bubbles, move at a higher velocity than those segments far away from the collapsing bubbles (Fig. 20.2). Thus, the polymer chain becomes elongated and this finally leads to chain scission (Cravotto and Angew 2007; Caruso et al. 2009).
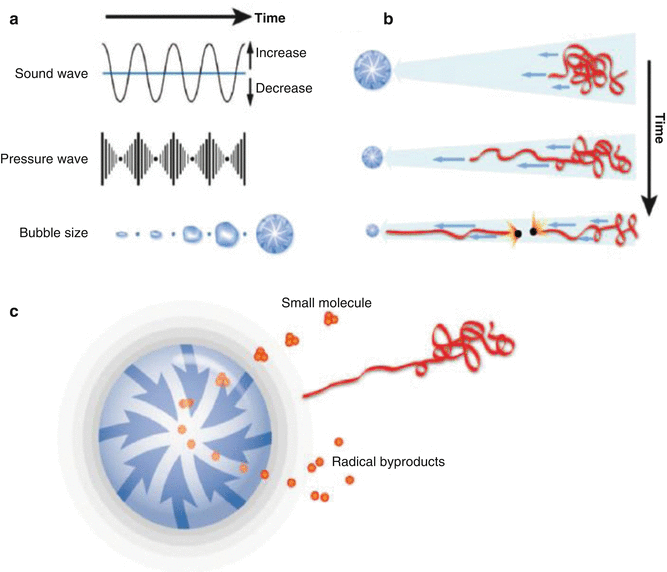
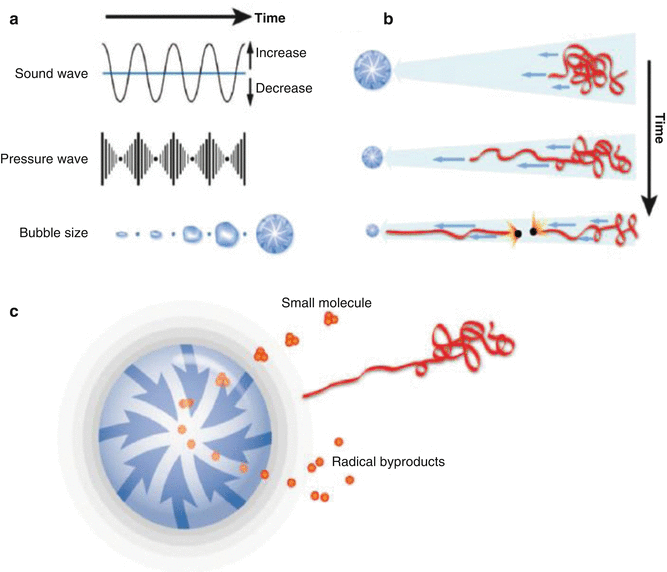
Fig. 20.2
Mechanism of ultrasound-induced polymer chain scission. (a) Gradual bubble formation; (b) Rapid bubble collapse generates solvodynamic shear to make polymer chain breaks; (c) Small molecules undergo pyrolytic cleavage to form radical byproducts (Cravotto and Angew 2007)
20.2.3.2 Ultrasound-Initiated Polymerization
The use of ultrasound to initiate the bulk or solution polymerization of monomers has been reported. Kruus and Price et al. successfully produced poly(methyl methacrylate) (PMMA) and proved that monomers could decompose to free radicals to initiate polymerization (Kruus and Patraboy 1985; Price et al. 1992). In addition, Makino et al. (1983) reported that water molecules were dissociated to high concentrations of OH · and H · radicals under ultrasonic irradiation. Henglein (1954) produced poly(acrylonitrile) in an aqueous solution in this way. By combining the advantages of sonochemistry and emulsion polymerization together, ultrasonically-initiated emulsion polymerization of methyl methacrylate, styrene and butyl acrylate/vinyl acetate has been reported (Liu et al. 1994; Chou and Stoffer 1999; Biggs and Grieser 1995; Xia et al. 2002). Compared with ultrasonically-initiated bulk or solution polymerization, ultrasonically-initiated emulsion polymerization can produce faster polymerization, higher monomer conversion and higher molecular weight polymers. Moreover, due to the intense dispersion, emulsifying and disrupting effects of ultrasound waves, nanoscale latex particles can be produced. This technique provides an alternative route to undergo mini- and micro-emulsion polymerization to produce polymeric nanoparticles (Zhang et al. 2002; Xia et al. 2001).
20.2.3.3 Ultrasonic In-situ Polymerization
Ultrasonic in-situ polymerization technology is a new technology to prepare polymer nanocomposites containing inorganic nanocomposites. By taking advantage of the multiple effects of ultrasound, i.e., dispersion, pulverizing, activation and initiation, the aggregation and entanglement of inorganic nanoparticles in aqueous solution can be broken down. At the same time, in-situ monomer polymerization of inorganic nanoparticles occurs on the surface. Consequently, the inorganic nanoparticles are coated by the formed polymer. Using ultrasonic in-situ polymerization, polymer-SiO2, polymer-Al2O3, polymer/carbon nanotubes, polymer-TiO2, polymer-Fe3O4 and polymer-montmorillonite nanocomposites were prepared (Xia and Wang 2002, Xia and Wang 2003, Xia et al. 2001, 2003; Wang et al. 2001, 2005; Qiu et al. 2007). This method has the following advantages: (i) Use of aqueous media, (ii) ease of operation and (iii) ability to design the encapsulated polymer layer to meet different requirements.
20.2.3.4 Site-Specific Ultrasound Degradation
Site-specific ultrasound degradation is a process in which degradation occurs at well-designed sites among polymer chains. The polymer normally contains a labile group, which can be broken under ultrasonic irradiation, initiating structural and property changes. This is also defined as mechanochemistry, and the reactions are much dependent on the molecular weight of the polymer, i.e., there exists a minimum molecular weight for the chain scission. In 2007 and 2009, Moore and colleagues proposed the mechanophore concept (Hickenboth et al. 2007, David et al. 2009). They introduced the mechanophore, such as benzocyclobutene, spiropyrans (SP) or dicyanocyclobutane, into PEG and poly (methyl acrylate) PMA chains. They found that ultrasound irradiation can induce structural conformation change, or site-specific degradation, by exerting the mechanical force on these mechanophore groups (Brantley et al. 2013; May and Moore 2013; Wiggins et al. 2013; Brantley et al. 2012; Ariga et al. 2012; Kryger et al. 2010). Such ultrasound-sensitive polymers are expected to have many potential applications, such as self-healable materials. Several distinguished research groups, including Moore, Craig, Bielawski and Sijbesma, are very active in this research field. The ultrasound mechanoresponsive materials can basically be divided into four kinds according to the research group: (1) Moore et al.: Benzocyclobutene (BCB) based PEG-BCB-PEG, spiropyran (SP)-based PMA-SP-PMA, dicyanocyclobutane(DCCB)-based PMA-DCCB-PAM; (2) Craig et al.: Gem-dichlorocyclopropane (gDCCs)-based poly(1,4-butadiene) PBD-DCC, (3) Bielawski et al.: Oxanorbornene Diels-Alder bond-based PMA-DA-PMA; 1,2,3-triazole-based PMA-triazole-PMA (Fig. 20.3); and (4) Sijbesma et al.: Bis(N-heterocyclic carbene) metal ligand poly(tetrahydrofuran). These researches are highly innovative and the molecular designs are elegant. However, some challenges remain: (1) The ultrasound power was too high: ~100 W, intensity ~10 W.cm−2, frequency 20 kHz; (2) The sonication time was too long, ~2 h; (3) The used organic solvent, like acetonitrile, is not environment-friendly, limiting the application to some degree. More rapid responsive mechanophores are still needed to be developed. On the other hand, Xia and Zhao focused their efforts trying to develop sensitive mechanophores in aqueous solution. They introduced the block copolymer with its micelle mechanophore concept, and established a new mechanochemical way to break the copolymer micelle. This has potential applications for controlled drug release (Wang et al. 2009; Zhang et al. 2009; Li et al. 2010).
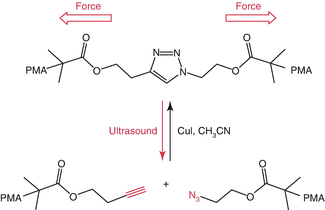
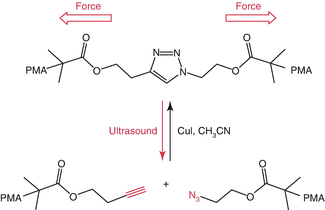
Fig. 20.3
Ultrasound-induced site-specific cleavage of 1,2,3-triazole mechanophore-containing polymers (Brantley et al. 2011)
20.2.4 High Intensity Focused Ultrasound (HIFU)
HIFU refers to the high frequency focused ultrasound of ultrasound beams that are emitted from a specially designed and curved transducer or phased arrays. The HIFU ultrasound beams are focused to a very small volume of several millimeters, which makes HIFU a more promising trigger for polymeric micelle drug delivery systems. At the focused spot, the ultrasonic intensity is very strong, while the intensity is very weak at other areas. A typical HIFU system consists of a signal generator, a power amplifier and an acoustic lens transducer. The signal generator controls the frequency and initial amplitude of the input signal, which is amplified using the power amplifier. The amplified signals are transmitted to the HIFU transducer to generate the desired ultrasound beam. They are constructed to make ultrasound beams converge and deposit maximum acoustic energy into the focal millimeter-sized volume. The acoustic lens transducer can determine the focal dimensions by the geometry of the transducer (aperture and focal length) and its operating frequency. The first report of HIFU application in humans was in 1960 (Fry and Fry 1960). However, this technique did not gain significant clinical acceptance until the 1990s, despite successful ophthalmological treatments before that date. In recent years, HIFU treatment has been increasingly used as a non-contact and remote control approach in medical treatment.
Compared with other techniques, such as cryotherapy, laser, microwave and radiofrequency, HIFU has some distinct advantages (Dogra et al. 2009). It is noninvasive and nonionizing, which means it can be repeated many times because it has no long-term cumulative effects. Due to the viscoelastic characteristics of human tissues, acoustic energy is lost and converted to heat; HIFU treatment can raise the focal area tissue temperature in seconds, and can maintain this temperature for 1 s or longer (Haar and Coussios 2007). Ultrasonic energy absorption within the focal volume induces high temperatures that can also be focused precisely on tissue volumes as small as several cubic millimeters, with temperatures outside this region being kept at noncytotoxic levels. This important HIFU lesion features makes the damage spatially confined, without damaging the intervening or surrounding tissue. Since the focal dimensions can be determined by the acoustic lens transducer, the desired size and shape of a larger HIFU target can be achieved by multiple sonications, combining individual lesions in a matrix format. Nowadays, a challenge in designing an optimal drug delivery system is the targeted, controlled and enhanced drug release in targeted areas. Compared to other stimuli, HIFU could be an ideal trigger to solve this issue because it can be focused and its parameters can be tuned in a remote way.
20.3 Micelles
Traditional surfactants include a hydrophilic head group and a hydrophobic long chain alkane. It can self-assemble into micelles in aqueous solution when the concentration is above critical micelle concentration (CMC). Similar to traditional surfactants, the polymer micelle is formed by self-assembly of amphiphilic copolymers, which include at least one hydrophilic block forming the shell, and at least one hydrophobic block forming the core. The polymer micelle diameter is about 10–100 nm. As a kind of smart material, stimuli-responsive amphiphilic copolymer micelles are of great interest, and have been extensively investigated as a delivery system for biomedical applications. This type of nanocontainer can encapsulate various poorly water-soluble pharmaceuticals in the hydrophobic core, and can be soluble or dispersible due to the hydrophilic corona. When polymeric micelles get into the human circulatory system, drug-containing micelles will accumulate at tumor cells by the enhanced permeability and retention effect.
For normal polymeric micelles without stimuli-responsiveness, drugs can only be released by diffusion and/or slow degradation of polymer materials; the responsive and release behavior of these micelles are usually uncontrollable. The stimuli-responsive polymeric micelles are of particular interest as they may have the following three advantages: (a) Better solubilization of hydrophobic drugs; (b) prolongation of the drug circulation time; (c) the release of guest molecules in a controlled way by external stimuli. Generally, hydrophobic drugs in the micelle can be released in response to a variety of stimuli, such as pH change,(Su et al. 2011; Zhou et al. 2011), temperature change (Eissa and Khosravi 2011, Liu et al. 2011), exposure to light (Knezevic et al. 2011; Yan et al. 2011) or enzymes (Coll et al. 2011; Pritchard et al. 2011). Ultrasound, which possesses the strong penetration effect and remote controllability, is a promising tool and attracts lots of interest as one kind of stimulus for polymeric micelle drug delivery systems. In recent years, there has been growing interest in using ultrasound as a stimulus to induce the disruption of copolymeric micelles and trigger the release of payloads (Geest et al. 2007; Geers et al. 2011; Pitt et al. 2004; Lensen et al. 2011).
20.4 Ultrasound-Mediated Polymeric Micelle Drug Delivery
Ultrasound is known as a powerful physical modality for spatial and temporal control of drug delivery. Ultrasound can effectively penetrate deep into the interior of the body in a noninvasive way. Fellinger and Schmid (1954) reported that ultrasound could enhance the percutaneous absorption of drugs, i.e., phonophoresis or sonophoresis. Skauen and Zentner (1984) proposed the concept of phonophoresis. Kost et al. (1989) firstly proposed the concept of releasing drugs entrapped in a solid polymer matrix through ultrasonic polymer degradation and erosion. Recently, ultrasound-triggered release from liposomes (Lin and Thomas 2003), polyelectrolyte microcontainers (Shchukin et al. 2006), multilayered capsules (Skirtach et al. 2007), microemulsions (Lee et al. 2008) and micelles (Rapoport et al. 1999; Husseini et al. 2000, 2002a, b, c; Husseini and Pitt 2008; Marin et al. 2002; Smith et al. 2008; Zhang and Pitt 2006) have been widely investigated. For ultrasound-mediated micellar drug delivery, the pioneer work was done by Pitt, Rapoport, Husseini et al. They first introduced ultrasound to control the drug release from polymeric micelles mainly by using low frequency ultrasound. The release mechanism is based on the physical disruption of the micelle and reversible release of payload. Xia and Zhao proposed the concept of ultrasound mechanochemically responsive polymeric micelles for drug delivery, mainly using high intensity focused ultrasound (HIFU). The release mechanism is based on chain scission, chemical disruption of the micelle and irreversible release of payload. Other groups include Deckers et al. (2013), Ugarenko et al. (2009), Myhr and Moan (2006) and Hasanzadeh et al. (2011).
20.4.1 Ultrasound-Triggered Physical Breakdown of Micelle and Reversible Release of Payload
In 1997, Rapoport and Pitt investigated ultrasonic-activated drug delivery from Pluronic P-105 polymeric micelles. They found the combination of ultrasound and micellar drug carriers can lower the effective dosage of an anti-cancer drug and reduce the toxic side effects associated with high doses of chemotherapeutic drugs (Munshi et al. 1997). Therefore, they established a novel modality for drug delivery and targeting, i.e., encapsulating the anti-cancer drug in micellar carriers, and then focusing ultrasound on the tumor site for controlled drug release. Since 1997, Rapoport, Pitt and Husseini et al. have carried out many investigations on ultrasound-mediated micellar drug delivery, which are summarized in some comprehensive reviews (Pitt and Husseini and Kherbeck 2013; Husseini and Pitt 2008, Sirsi and Borden 2014, Kiessling et al. 2014). In their research, the ultrasound-triggered release mechanism is “physical”, and can be described as follows: The micelle is disrupted or disassembled under ultrasound perturbation resulting from ultrasonic cavitation or mechanical effect, and the payload is released from the micelle. Once the ultrasound ceases, the micelle can be reassembled again, and the payload can be re-encapsulated. For this mechanism, the micelle disruption is temporary and the drug release is reversible.
The work of Rapoport, Pitt and Husseini et al. is not only pioneered, but also comprehensive. The effect of ultrasound factors, including ultrasound intensity, low or high frequency, pulsed or continuous wave, were examined, and also the ultrasound triggered release kinetics and mechanism were investigated in-depth. For the micelle carrier, they mainly used the FDA-approved Pluronic micelle, composed of triblock copolymer poly(ethylene oxide)-poly(propylene oxide)-poly(ethylene oxide) (PEO-PPO-PEO), or stabilized Pluronic micelle by crosslink or interpenetrating network. Most importantly, they did a lot of excellent work on ultrasound-triggered in-vitro drug (mainly doxorubicin) release to different cancer cells, such as promyelocytic leukemia HL-60, MDR ovarian carcinoma and breast cancer cells MCF-7, A2780, MDA-MB-231. Additionally, they studied in-vivo drug release in animal models, such as rat hind leg with a colon carcinogen DHD/K12/TRb tumor cell, and mouse with colon or breast cancer. All in-vitro and in-vivo experimental results were positive. Under action of the new modality, i.e., ultrasound-mediated micellar drug delivery, the cellular drug uptake was improved, and consequently cancer cells were destroyed and tumor reduction was significant.
Husseini et al. investigated the factors affecting acoustically-triggered drug release from polymeric micelles (Husseini et al. 2000). Real-time fluorescence detection was used to measure the drug release from Pluronic P-105 micelles under continuous wave or pulsed ultrasound in the frequency range of 20–90 kHz. Two fluorescent drugs (Doxorubicin and Ruboxyl) were used. The drug release was found to decrease at higher frequencies, indicating an important role for transient cavitation in drug release. The release of doxorubicin (DOX) was higher than that of Ruboxyl due to stronger interaction and deeper insertion of Ruboxyl into the core of the micelles. Drug release was higher at lower Pluronic concentrations, and increased with increasing power density. At constant power density, peak release under pulsed ultrasound, with duration longer than 0.1 s, was the same as that under continuous ultrasound. Released drug was quickly re-encapsulated between the ultrasound pulses, suggesting the non-extravasated and non-internalized drug would circulate in the encapsulated form upon ultrasound halts, thus preventing unwanted drug interactions with normal tissues. Marin et al. also studied the effect of a continuous wave and pulsed 20-kHz ultrasound on DOX uptake by HL-60 cells from phosphate buffered saline solution (PBS) and Pluronic micellar solutions (Marin et al. 2001a, b). Under both the continuous wave and pulsed wave ultrasound, DOX uptake from PBS and Pluronic micelle solutions can be enhanced. Drug uptake can be further enhanced under higher ultrasound power, accompanied by extensive cell sonolysis. The drug uptake also increased with increasing pulse duration in the range 0.1–2 s. Over 2 s pulses, the uptake was similar to that under continuous-wave (CW) ultrasound. At an ultrasound frequency of 20 kHz and power density of 58 mW/cm2, no significant drug release was observed when exposed to ultrasound for less than 0.1 s. This shows a threshold time value. Above this threshold, the amount of release was shown to increase as the pulse length increased up to 0.6 s. Under ultrasound treatment, micelles were destructed because of the shock waves produced by collapsing bubbles, inducing the release of hydrophobic drugs. After the ultrasound treatment halted, micelles could be reassembled and drugs could be re-encapsulated.
Regarding the effect of ultrasound frequency on polymeric micelle drug release, there is no doubt that low frequency is more effective than high frequency. However, there are some inconsistent results. In 2002, Marin et al. observed a DOX release of ~8.5 % at a power density of ~7 W/cm2 under high frequency 1 MHz ultrasound (Marin et al. 2002). On the other hand, Diaz de la Rosa (2007) found no DOX release occurs at 500 kHz high frequency ultrasound, even at an ultrasound intensity of 20 W/cm2. It should be mentioned that they didn’t investigate the effect of HIFU on drug release. Kobayashi et al. (2012) investigated the release of hydrophobic dye from Pluronic micelles under low frequency ultrasound. They also found the hydrophobic dye is more easily released under lower frequency ultrasound. Compared with the thermal effect, mechanical cavitation is more significant.
Husseini et al. (2002a, b, c) measured the kinetics of acoustic release of DOX from and subsequent re-encapsulation of DOX in Pluronic P105 micelles. Several physical models and their corresponding mathematical solutions were analyzed to see which model most closely fitted the data. The model of zero-order release with first-order re-encapsulation appears to represent data from this polymeric system better than other models.
Stevenson-Abouelnasr et al. (2007) investigated the release mechanism and release kinetics of DOX from Pluronic P105 micelles during ultrasonication and its subsequent re-encapsulation upon cessation of insonation. The mechanisms included micelle destruction, destruction of cavitating nuclei, reassembly of micelles and the re-encapsulation of Dox. The micelles are destroyed because of cavitation events produced by collapsing nuclei. The slow destruction of cavitating nuclei results in a slow partial recovery phase when a small amount of Dox is re-encapsulated. The reassembly of micelles and the re-encapsulation of Dox are independent of ultrasound. Parameters for the model were determined based upon the best-observed fit to experimental data.
Concerning the drug release mechanism from Pluronic micelles, there is no doubt that ultrasound indeed physically breaks the polymer micelle. However, it is not so clear how the ultrasound breaks the micelle. In other words, what is the interaction mechanism between the micelle and the ultrasound wave? Cavitation or mechanical vibration? Marin et al. (2002) studied the relationship between ultrasound intensities and DOX release, as well as the relationship between the radical concentration and ultrasound intensity. They found that for 10 % DOX drug release from Pluronic micelles, the required ultrasound intensities are: 20 kHz: 0.058 W/cm2; 67 kHz: 2.8 W/cm2; 1.0 MHz: 7.2 W/cm2. However, the radical trapping experiments showed that the radial formation thresholds under ultrasound were: 20 kHz: 0.08 W/cm2; 67 kHz: 1.0 W/cm2; 1.0 MHz: 3.6 W/cm2. This result implies that the DOX release was not directly related to the ultrasonic cavitation, which can be characterized by radical formation. Husseini et al. (2005) re-investigated the role of cavitation in acoustically-activated drug delivery. The acoustic spectra were collected and analyzed at the same spatial position as fluorescence data to explore the role of cavitation in drug release. The results showed a strong correlation between percentage drug release and subharmonic acoustic emissions, and the drug release was attributed to the collapse cavitation that perturbs the structure of the micelle and releases drug. Since then, the mechanism of cavitation for micelle disruption was widely accepted.
Regarding the carrier design, Pitt, Rapoport and Husseini mainly focused on Pluronic micelles. However, they also made some valuable modifications on the carrier system. One disadvantage for P-105 micelles is that they are not very stable under dilution. Rapoport et al. (1999) designed three routes to overcome this problem: (1) Radical crosslinking of micelle cores; (2) introducing vegetable oil into the Pluronic solutions, and (3) polymerization of the temperature-responsive LCST hydrogel in the core of Pluronic micelles, called Plurogel. They confirmed the third route is the best one, and found that the ultrasonication actually enhanced intracellular drug uptake from dense plurogel micelles.
Husseini et al. (2002a, b, c) prepared P-105 micelles stabilized with an interpenetrating network of N,N-diehtylacrylamide NanoDeliv (TM). The results showed that 2 % DOX can be released within 2 s from the stabilized micelles under ultrasound at 70 kHz, which was not significantly different from unstabilized P-105 micelles. The drug re-encapsulation upon insonation cessation was also complete. In 2007, they measured the release of DOX from unstabilized Pluronic 105 micelles, Pluronic P105 micelles stabilized with an interpenetrating network of N,N-diethylacrylamide and poly(ethylene oxide)-b-poly (N-isopropylacrylamide)-b-poly(oligolactylmethacrylate) micelles with stabilized cores. It seemed that they obtained the opposite conclusion to the previous experiment, i.e., the DOX release from unstabilized Pluronic micelles is much greater than that from the stabilized and crosslinked micelles, however the onset of release occurs at the same ultrasonic power density for the three kinds of carriers investigated (Husseini et al. 2007). In 2009, they found that ultrasound at frequencies 70 and 476 kHz, with a mechanical index of 0.9, can disrupt stabilized micellar covalent networks (NanoDelivTM), but the network degradation time constant is very long compared to the time constant pertaining for drug release from micelles. After exposure to 70 kHz and 476 kHz ultrasound for 1 h, no significant difference between the network degradation was observed (Husseini et al. 2009).
Zeng and Pitt (2006) prepared a polymeric micelle system with a hydrolysable segment for drug delivery. This micelle is composed of an amphiphilic copolymer, poly(ethylene oxide)-b-poly(N-isopropylacrylamide-co-2-hydroxyethyl methacrylate-lactaten), and has a half-life of about 48 h at 40 °C. The DOX release was ~ 4 % at body temperature under ultrasound, and the drug was returned to the polymeric micelles when insonation ceased. Smith et al. (2008) confirmed that high intensity focused ultrasound can trigger drug release from the pH responsive PEGylated micelles and can improve drug uptake by H69 human carcinoma cells in-vitro. Husseini et al. (2013) reported the use of the Pluronic P105 micelles with a folate-targeting moiety containing DOX, and investigated the acoustic release of the drug. The maximum amount of release reached ~ 14 % at an ultrasound power density of 5.4 W/cm2. The novel, well-designed folated Pluronic micelle, combined with external ultrasound stimulus, can reduce the adverse side effects of chemotherapy by preferentially and actively targeting tumor cells.
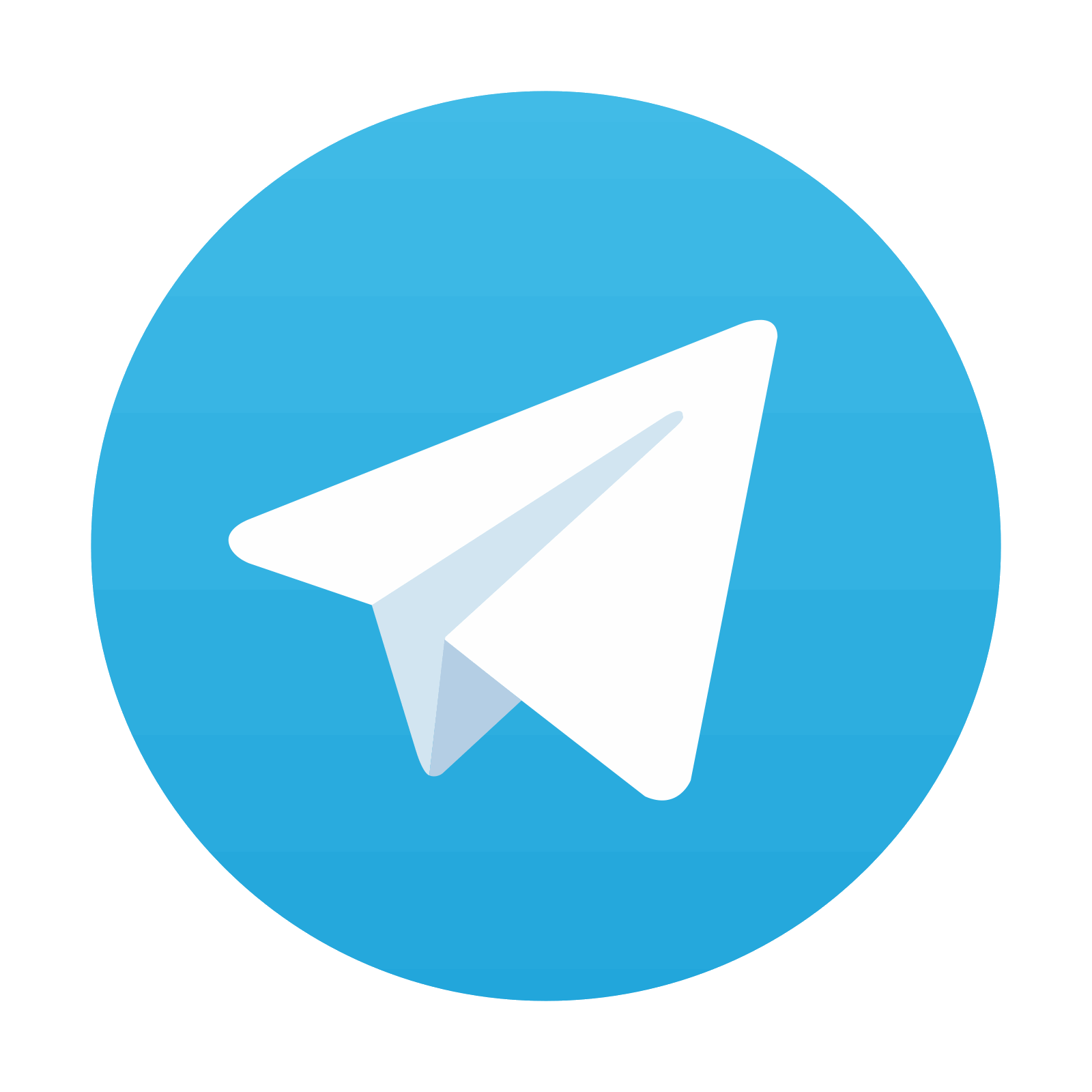
Stay updated, free articles. Join our Telegram channel
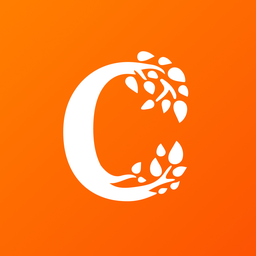
Full access? Get Clinical Tree
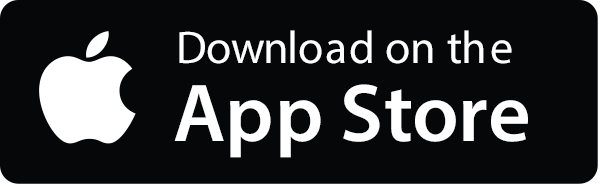
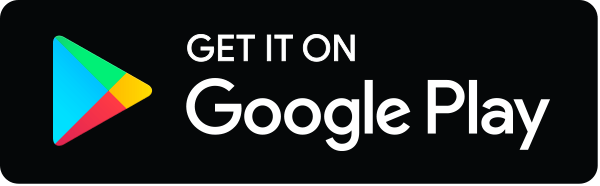