Introduction In this chapter, osteo-articular and soft tissue injuries of the cervical spine are systematically addressed with the benefit of postmortem and experimental pathological findings. The underlying biomechanical aspects of the resultant injuries need to be appreciated as they form the foundation of correct image interpretation. At the same time the reader realizes that the vast spectrum of spinal injury poses significant challenges to the imaging modalities currently available for the diagnosis and exclusion of injury. Fractures of the occipital condyle have long been regarded as rare injuries to the atlanto-occipital joints. Less than 20 such clinical cases are discussed in the literature, from Bell’s initial description of the injury in 1817 through to the mid-1980s.1–4 In contrast, condylar fractures in fatal trauma are a familiar topic in forensic medicine.5–9 Alker et al and Saternus each cite an incidence of 0.6 % in the setting of fatal trauma.5,7 Recent radiological reviews give an incidence of occipital condylar fracture of up to 16 % of patients with craniocervical injury.10–13 The attention given to the alar ligaments in whiplash injuries has also increased clinical interest in the traumatology of condylar fractures. Table 2.1 lists the mechanisms of injury for a condylar fracture.14 This does not include the horizontal avulsion fracture6 because such a mechanism of injury in a normal joint with negligible friction would not represent plausible explanation.15 The most commonly used classifications of occipital condylar fractures are based on radiological criteria.16,17 The most useful one is that of Leone and coworkers, which includes multiple new features.12 Using a detailed review these authors point out that the clinical symptomatology of condylar fractures is so inconsistent that the diagnosis is rarely made clinically and requires dedicated cranio-cervical CT assessment. Fig. 2.1 Oblique radiographs of postmortem cervical spine showing complete atlanto-occipital dissociation (arrows). Transmission of the force to the base of the skull via the mandibular joints. Fig. 2.2 Incomplete disruption of the occipital condyle with hemorrhage and fragment formation in the region of the insertion of the alar ligament (arrow). In our own material, including over 2000 studies of fatal trauma, traction injuries in the form of condylar avulsion warrant a special mention among those constellations that lead to condylar fracture. Figure 2.1 shows an example of a complete insertion avulsion, and Figure 2.2 shows an example of an incomplete one.
Atlanto-Occipital and Atlanto-Axial Joints
Condylar Fracture
Traumatology
Biomechanics
1. Axial compression | (Jefferson type) condylar impression |
2. Axial traction | (Hangman’s type) condylar retraction |
3. Rotation with axial loading | Condylar retraction |
4. Oblique compression | (Burst fracture of the abutment) frontal condylar fracture; contralateral |
5. Oblique traction | (Horizontal shear force) a skull base fracture as in a contralateral horizontal fracture |
6. Transverse shear force | (Longitudinal fracture of the base of the skull) partial condylar avulsion |
Compression of the skull in the sagittal plane initially causes elastic deformation of the base of the skull. Compression exceeding the threshold value causes the bone to burst, producing transverse shear stress. The result is a sagittal fracture of the base of the skull. This transverse shear stress is resisted by a particularly stable structure at the base of the skull. This consists of the double-ring structure of the margin of the foramen magnum together with the occipital condyles and the atlas. The two rings are additionally stabilized by sturdy ligament structures (alar ligaments and the transverse ligament of the atlas) that provide stability against transverse shear stresses. A fall or blow that shatters the skull exerts traction on this exceedingly sturdy structure. This type of traction injury of the bone-ligament interface takes the form of avulsion of the insertion of the alar ligament and a fragment of variable size from the occipital condyle. Figure 2.2 demonstrates that such an insertion avulsion will not necessarily be complete.
Alar Ligaments
Topography
Many authors concur in specifying that the alar ligaments course superiorly from the odontoid process to the condyles. In contrast, Dvorac et al demonstrated significant variability in the course of the ligament in their material.18–21 In one quarter of their cases, the alar ligaments were observed to course inferiorly from the condyle. From our group, Thrun performed dissections on forensic material (n = 31) to pursue this line of inquiry.22 Those dissections confirmed this variability. The ligaments normally insert into the upper twothirds of the lateral surfaces of the odontoid process. However, individual fibers may also insert into the apex of the odontoid process. In this study too, the ligaments in one quarter of the cases coursed inferiorly from the odontoid process to the occipital condyles. Typically (in 75 % of these cases), a portion of the most superior fibers passed over the apex of the odontoid process. The anterior bone spur frequently observed with the steep type of odontoid process (kyphotic odontoid process as described by Krmpotic-Nemanic and Keros) limits the anterior extent of the structure.23
In over one-third of the cases, the ligaments were multipartite, specifically consisting of two to five parts. In this context, we should also mention that a distinct portion between the condyle and atlas also occurs.24
The fan-shaped divergent angles of the alar ligaments (both to the condyle and to the odontoid process) in our own study group are significant from a functional standpoint in that they further restrict rotation in addition to the normal anatomical limitation. Such configurations occurred in 15% of the cases studied. Here, the fiber bundles were unilaterally or bilaterally twisted anteriorly toward each other.
Biomechanics
The findings presented indicate that the alar ligaments should not be regarded as compact, homogeneous ligaments and that their course may vary between individual patients. Contrary to the majority opinion expressed in the literature, it is not even true that the fibers of the structure course superiorly from the odontoid process to the condyles in most cases.
Two conclusions may be drawn from this. First, partial ruptures of the alar ligament are indeed possible; second, injury is not due solely to the unphysiological rotational stress. On the contrary, in any given injury, the patient’s specific anatomical constellation is not predictable and cannot be visualized with currently available imaging modalities.
Traumatology
We have investigated in our postmortem study injuries of the alar ligaments in fatal trauma.25 Fatal trauma can occur without any involvement of the cervical spine. Conversely, findings included every stage from lack of morphological evidence of injury to massive fracture dislocation or rupture with dislocation. Alar ligament injuries were categorized according to a semi-qualitative five-stage classification system. The individual classes do not represent a uniform distribution; there are gaps in the intermediate stages. However, the respective incidence of uninjured, moderately injured, and severely injured spines were in a comparable range.
As expected, the results demonstrate a correlation between the overall severity of the injury to the cervical spine and the extent of injury to the alar ligaments. The alar ligaments were injured in one-third of all cases of fatal trauma.
In three of 30 cases, the sole injury to the cervical spine and atlanto-occipital and atlanto-axial joints consisted of injury to the alar ligaments in the form of hemorrhage.
Most cases of massive cervical spine trauma also involved rupture of the alar ligaments. However, this was not a constant finding. With fractures of the odontoid process, findings in our own material included ligament hemorrhages but not complete ligament ruptures. Avulsion of the ligaments from the odontoid process occurred primarily in rotation. This correlation with rotation was not as clear in experimental postmortem studies of anatomical specimens obtained from patients who had stipulated that their bodies could be used for scientific research after death.
Cruciform Ligament, Apical Ligament, Anterior Atlanto-Occipital Membrane, and Anterior Longitudinal Ligament
Anterior Atlanto-Occipital Membrane and Anterior Longitudinal Ligament
As a result of traction, the anterior longitudinal ligament is injured twice as often as the anterior atlantooccipital membrane. This means that the anterior and posterior conditions are identical. In this regard, it should be noted that only traction, not compression, can lead to ligament injury.
Apical Ligament
Although they rarely receive clinical attention, injuries to the apical ligament are also common. A remnant of the corda dorsalis, this slender ligament courses with a small artery and veins between the clivus and the apex of the odontoid process. Although the ligament bears physiological stresses in flexion and extension,4 it does not serve any significant functional purpose in the atlanto-occipital joint. It can rupture in combination with other soft tissue injuries. However, traumatic injury is usually only indirect (Fig. 2.3) and involves hemorrhaging from injured accompanying blood vessels.26 Accordingly, an isolated injury to this structure is not detectable even with current imaging modalities. An important diagnostic aspect is that even bony injuries (avulsion of the insertions) practically never occur because of the small size of the ligament.
Cruciform Ligament
Rupture of the vertical portion of the cruciform ligament indicates an extensive injury. With axial traction or flexion, it often tears along with the dura, which is usually also separated from the inner surface of the adjacent skull base.
An interrelated complex of ligament rupture and ring fracture of the skull base characterizes massive traction injuries. Figure 2.4 shows an example of a marginal clivus avulsion.
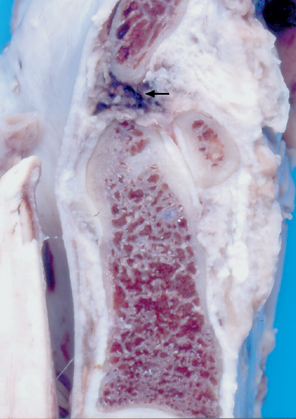
Fig. 2.3 Circumscribed hemorrhage around the apical ligament of the odontoid process due to a direct traction injury at the craniocervical junction (arrow).
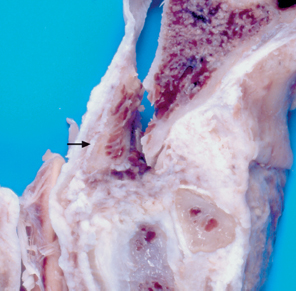
Fig. 2.4 Bone fragment (arrow) avulsed from the clivus by traction secondary to a ring fracture of the base of the skull.
Forces acting on the skull can fracture the skull base in a circular pattern emanating from the center of the injury. When this occurs (such as in a fall on the back of the head), a narrow rim is often avulsed from the clivus.9,27–29 In cases such as a fall on the central portions of the skull or on the heels or buttocks, there is a wedge of compression pointing inward into the cranial cavity while the traction produces a fracture cone that becomes wider as one moves distally.
In forced flexion of the skull, the posterior arch of the axis acts as a lever to transfer the force of injury via the transverse ligament of the atlas to the posterior surface of the odontoid.30 This can produce an odontoid fracture or a rupture of the horizontal part of the transverse ligament. Several systematic studies of the strength of the ligament have been conducted and included in the analysis of the injury.31,32 This has shown that severe regressive changes in the ligament occur with increasing age. These changes are conducive to ruptures. Our own experimental studies of tearing of the transverse ligament of the atlas have demonstrated that in over half of all cases, fine avulsion of the insertions from the lateral masses had occurred (Mann, unpublished) (Fig. 2.5). There are only a few descriptions of axial avulsion of the skull with the atlas in the absence of a rupture of the transverse ligament of the atlas or odontoid fracture.7
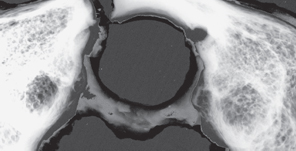
Fig. 2.5 Montage of a soft tissue radiograph superimposed on the osseous structure (C1) in a case of an experimentally induced tear of the transverse ligament of the atlas from the right lateral mass.
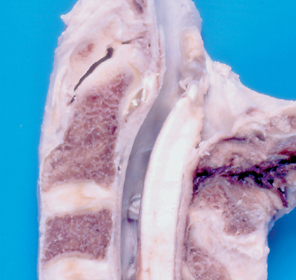
Fig. 2.6 Rupture of the posterior atlanto-axial membrane in flexion trauma.
Posterior Atlanto-Occipital Membrane and Tectorial Membrane, Epidural Bleeding
The most common injuries to the occipital and cervical region are ruptures and hemorrhaging deep to the posterior ligament structures. Disregarding hemorrhaging into the posterior atlanto-occipital membrane from a skull fracture (a common indirect injury), our own early studies have shown that about half as many injuries occur in the next layer, the tectorial membrane (Fig. 2.6).33 The reason for this is the difference in strength and size of the two structures; the posterior atlanto-occipital membrane is significantly stronger than the tectorial membrane.
This means that the typical form of mechanical stress leading to rupture of the tectorial membrane occurs when the posterior arch of the atlas and the spinous process of the axis are drawn apart with the head in forward flexion. In flexion, the posterior arch of the atlas is carried along by its strong ligamentous attachment to the skull. Accordingly, a complete or partial tear of the tectorial membrane is invariably an associated injury with an odontoid fracture from flexion trauma.
This is why it appears strange that injury to the tectorial membrane occurs in the presence of stress in the opposite direction, namely with an odontoid fracture in extension trauma. Yet this too is a constant finding. This may be explained by translation, which also produces an unphysiological stress sufficient to cause a rupture. However, most actual accidents leading to injury of the tectorial membrane involve complex rotational motions.
It would be wrong to create the impression that injury to the tectorial membrane is typically associated with a bony injury. On the contrary, it is often observed as an isolated soft tissue injury to the cervical spine. Its level is determined by the size difference between the two posterior ligament systems extending between the occiput and C2. Posterior ligament injury is regularly accompanied by epidural bleeding. Epidural bleeding in the cervical spine is common in fatal trauma. The term fatal trauma could create the impression that the cervical spine is the site of the fatal injury. However, this tends to be the exception in those cases examined by coroners. On the contrary, every degree of involvement of the cervical spine may be observed, ranging from absence of injury to fatal spinal cord injury. Diffuse or circumscribed epidural bleeding may be observed with nearly every soft tissue injury to the cervical spine and with bony injuries. It most commonly occurs in the posterior epidural region.7,34,35
The structural differences between the posterior atlanto-occipital membrane and tectorial membrane suggest that they have different functions. At the suggestion of Dr. Nägerl (communicated personally), we are currently exploring whether these two ligament structures differ with respect to sensory elements such as lamellated corpuscles and Ruffini corpuscles. The thinking is that the posterior atlanto-occipital membrane may have more of a mechanical function whereas the tectorial membrane may also have a proprioceptive function.
Atlas Fracture
Anterior Arch
The older literature specifies the incidence of anterior arch fractures relative to posterior arch fractures as about 1:3. Several earlier postmortem studies have shown that this relation can be reversed in the case of fatal trauma,7 namely two to one. Avulsion of the insertions of the anterior longitudinal ligament with a fragment of varying size from the anterior arch of the atlas may occur in the common extension injuries. Another mechanism, namely horizontal shear forces that cause transverse fractures of the anterior arch,36 did not appear plausible in adults. However, in the case of an almost 2-year-old child described by Töndury and Tillmann where the odontoid and central anterior arch of the atlas were not yet ossified,37 both the avulsion through the cartilage of the apex of the odontoid, i. e., not at the bone-cartilage interface,38,39 and a bony avulsion of the inferior margin of the arch of the atlas may be attributed to a horizontal shear component.
In another example from our own studies, external flexion with extensive tearing of the posterior ligaments between the occiput and C2 led to a tear in the superior capsule of the median atlanto-axial joint and to bending stresses in the articular facet pressed against the anterior surface of the odontoid process. This resulted in a fracture in the margin of the median atlanto-axial joint (articular facet).
In combination with a basal odontoid fracture, such an avulsion of the inferior margin of the anterior arch of the atlas can also occur as asymmetric injuries. This has been demonstrated by an earlier study (Fig. 2.7).40
In this regard, it should be noted that in animal studies the ligaments between the occiput and C2 tend to rupture at the same kinetic energy in both extension and flexion trauma.41,42 This means that the more common injury is not the sagittal fracture of the anterior arch of the atlas but the horizontal fracture.
Furthermore, the presence of elastic structures even permits a symmetrical avulsion of the anterior arch of the atlas (Fig. 2.8). The example shows step-shaped avulsion of the unossified anterior arch in a nine-yearold subjected to compression in flexion (anteriorly and inferiorly directed force vector). This type of fracture would best be described as a Jefferson fracture, which would be open to question in an adult (see the section Jefferson Fracture below).
Jefferson Fracture
Traumatology
Since Jefferson’s description,43,44 the bilateral fracture of the anterior and posterior arch of the atlas is characteristically attributed to axial loading. Lateral avulsion of the lateral masses is widely accepted as the mechanical cause of the fracture. The thinking is that compression causes the atlas to break apart like two wedges under a horizontal shear stress. The occipital condyles form the superior compressing wedge, and the two superior articular facets of the arch of the axis form the inferior wedge.
The literature repeatedly mentions that Jefferson never succeeded in reproducing this type of fracture in experiments on human anatomical specimens even when extreme force was applied.
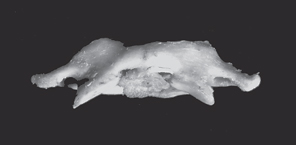
Fig. 2.7 Anterior view of C1 with a portion of the fractured dens. This shows a C1 horizontal fracture of the lower margin of the facet for the odontoid process to the left of the mid-line extending to the left lateral mass, due to a bending strain under a ventrally flexing vector (dens fracture).
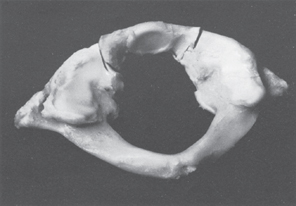
Fig. 2.8 Avulsion of the anterior arch of C1 that is incompletely ossified with the lateral masses, from an anteriorly and inferiorly directed vector force. The transverse ligament of the atlas is intact.
The section on the Biomechanics of Condylar Fracture above describes in detail how the ring structure of the atlas and the transverse ligament that balances shear forces give the atlas a high degree of dimensional stability. Horizontal shearing of the lateral masses would require either significant elastic or plastic deformation or rupture of the ligament. Earlier experimental tests of tensile strength suggest that stretching without structural damage under actual fracture conditions is highly improbable. Tears of this ligament structure in atlas fractures are also rare. However, one would expect them according to current thinking.
In a finite element model, axial compressive pressure induces high concentration of localised stress at the anterior and posterior arch of the atlas.45 These authors, however, did not include in their calculations the transverse ligament of the atlas which neutralizes tension.
This is sufficient cause to critically reexamine the assertion that Jefferson fractures are caused by axial compression.
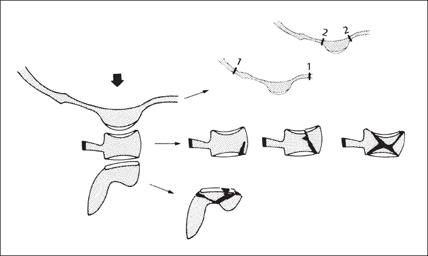
Fig. 2.9 Traumatic sequelae from craniocervical axial compression: fractures of the occipital condyles (see Figs. 2.1, 2.2), lateral mass of C1, articular surface of C2.
Biomechanics
Our line ofinquiry was to investigate whether the Jefferson fracture represents a model fracture for axial loading. Our working group performed fracture tests (n = 400) with a custom test stand of our own design.46,47 The skull base, an indestructible structure for test purposes, was made of metal; the test specimens representing the atlas and axis were identical plaster casts.
Two real objects of different types were used as models. As regards the position of the odontoid, we used one specimen inclined 1° anteriorly and one inclined 7° posteriorly, according to Krmpotic-Nemanic and Keros and Koebke.23,48 These authors refer to the anteriorly inclined type as kyphotic and the posteriorly inclined type as lordotic. Results of measurements of collected material and our own specimens have shown that the inclination of the odontoid’s axis is indicative of the overall configuration of the region from the occiput to C2. For example, a sturdy arch of the atlas with a wide articular facet is typical for the kyphotic odontoid, whereas a slender configuration is typical for the lordotic type.48 In terms of Jefferson’s model, this would correspond to wide, flat superior and inferior wedges in the case of a kyphotic odontoid whereas the adjacent structures would correspond to narrow wedges in the case of a lordotic odontoid.
The test specimens were subjected to loading at 10 different angles until the fracture occurred; the transverse ligament of the atlas was designed not to tear. The shape and localization of these fractures (initial fractures) were recorded. Then loading was continued with a defined force until a second fracture occurred.
Using this experimental design, we produced atlas fractures in 84% of all cases with a flat wedge and in 94% of all cases with a narrow wedge. These corresponded to fracture types that actually occur. However, no Jefferson fractures occurred in 20 experiments with purely axial loading whereas seven occurred with eccentric loading. The final results of the second fractures in 200 fracture experiments included 16 fracture patterns of the Jefferson type in addition to three related forms of symmetrical atlas fractures. In two of these cases, the fracture line coursed through the center of the articular facet; in the third case, it coursed through the anterior portions of the lateral masses. In the clinical literature, this latter case is regarded as equivalent to a Jefferson fracture.
Twelve of the subsequent Jefferson fractures resulted from less extensive initial fractures. With respect to the final results after the second fracture, we found that Jefferson fractures occurred almost twice as often with axial loading than with eccentric loading. We may, therefore, conclude that these fractures occur in one of two ways. The first and more common way would be as a subsequent symmetrical fracture of the anterior and posterior arches of the atlas secondary to destabilization of the ring of the atlas at any given site under axial loading. Applied to actual clinical conditions, this frequency distribution is probably the reason for previous notions about the etiology of this type of fracture.
The second way in which a Jefferson fracture occurs is due to a bending stress on the atlas in the sagittal plane, i. e., a nonaxial stress. In this regard, the question arises as to whether such a mechanical interpretation may correctly be derived from fracture tests on plaster models.
These experimental results for the Jefferson fracture are not isolated findings. Nor is it the case that homogeneous plaster is assumed to be equivalent to bone with its functional material distribution. Nevertheless, these fracture experiments provide information about the distribution of stresses in a three-dimensional model. Our fracture experiments can build on those performed by Jefferson himself. Additionally, they provide an explanation of why the transverse ligament of the atlas usually remains intact in a Jefferson fracture.
Lateral Mass
Jefferson himself noted that axial compression at three levels can lead to bony injury.43 Figure 2.9 illustrates the various different types of fractures. The occipital condyles represent the upper topographic level. They are usually affected in large-scale injuries in the setting of a ring fracture of the skull base, although a narrow avulsion can occur in the form of a local impression fracture (see the section Condylar Fracture).
The middle level is the level of the lateral mass and the lower one is that of the base of the joint of the axis, the superior articular facet.49
The compression fracture type is primarily attributable to force acting on the central portions of the skull. However, a fracture of the lateral mass can also occur indirectly as a result of the force of the weight of the skull as can occur in a fall on the lower extremities and the buttocks.50
Compression fractures of the atlas include direct and indirect injuries. Even Jefferson was familiar with direct compression fractures. He refers to Ludolff in this regard.43,51
Indirect fractures occur where a lateral stress acts on the skull above the center of gravity with compression, for example in a car accident where a passenger is thrown against the side column of the vehicle, with a resulting compression injury. These indirect fractures are the result of high compressive forces on the contralateral side. However, ipsilateral traction and contralateral compression, i. e., bending stresses on the atlas, produce oblique fractures far more often than compression fractures. Usually these bony injuries are attributable to complex rotational motions. These eccentric stresses lead to subluxation in the atlantooccipital joint, concentrating the load at a single point.
Incomplete avulsion of the cortex with the most anterior part of the articular surface of the lateral mass has been described.7 This is the rare finding of a floating subtotal dislocation. Most authors describe the bony injury to the lateral mass as medial avulsion of that structure.31,50,52–54 This type of fracture is regarded as an avulsion of the insertions of the transverse ligament of the atlas, which is difficult to imagine with an intact bony ring. Accordingly, Figure 2.10 attempts another mechanical interpretation based on our own early case studies.55 What had to be taken into consideration was that the transverse ligament of the atlas had remained intact while the medulla had suffered a massive shear injury. The axis and the odontoid in particular were intact.
On the basis of the fracture experiments mentioned above, we must proceed from the assumption of a Jefferson fracture with eccentric stress for our fracture analysis. This is because the findings are due to a massive extension trauma resulting from a fall from a height of 12 m on the left half of the forehead.
The oblique sagittal fracture surfaces passing through the anterior portions of the lateral masses can be explained by high compressive loads on the posterior portions of the superior articular facet with rotation around the coronal axis and collapse of the posterior arch of the atlas. This in turn represents a Jefferson fracture without the Jefferson effect. The literature includes descriptions of vertical avulsion of the anterior arch of the atlas in children without prior or concomitant fracture of the posterior arch of the atlas. Two such cases include a 2-year-old girl and a 9-year-old boy with a step-shaped avulsion in massive flexion trauma (see Fig. 2.8).56,57
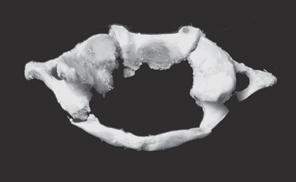
Fig. 2.10 Jefferson fracture without Jefferson effect. Note the course of the fracture bilaterally through the articular surfaces.
Posterior Arch
Symmetrical fractures of the posterior arch of the atlas are primarily attributed to bending stresses around a coronal axis, although Sköld emphasizes the rotational component.58
Flexion
In flexion trauma, the traction of the strong posterior atlanto-occipital membrane flexes the posterior arch of the atlas as the skull is flexed. This opens the adjacent segment (C1-C2) posteriorly (Fig. 2.6), which stretches and ruptures the tectorial membrane and causes epidural bleeding and tears in the adjacent musculature of the back of the neck. The inferior margin of the anterior arch of the atlas strikes against the anterior surface of the odontoid. This can create defects in the odontoid or avulse the cortex from the inferior margin of the median atlanto-axial joint (see Fig. 2.7).58 This horizontal shear force often leads to partial separation of the C2-C3 intervertebral disk. Complete separations are rare.
This section examines the typical bony injury, namely superior bending or fracture of the posterior arch of the atlas. The anterior arch can only be bent upward or twisted with the transverse ligament intact when the ring structure of the atlas has become unstable. The nature of the secondary injury depends on the direction of the force vector incident on the occiput and the distribution of material. Accordingly, this injury may take the form of a symmetrical fracture through the articular surface or bilateral arch fracture, or an asymmetrical injury with avulsion of the arch from the lateral mass or facet. These combined injuries to the arch of the atlas are common.
Extension
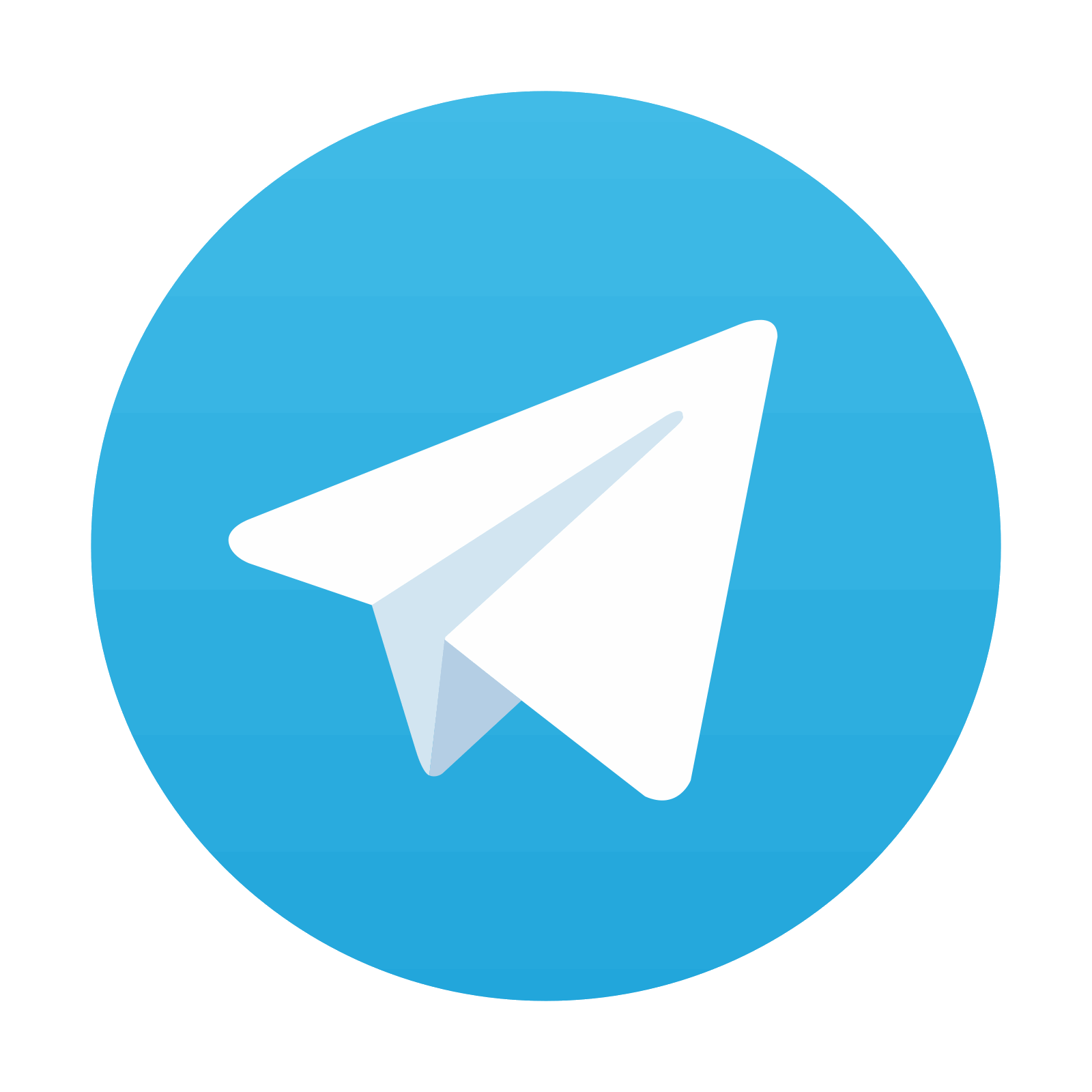
Stay updated, free articles. Join our Telegram channel
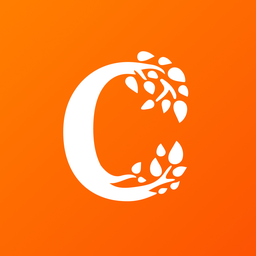
Full access? Get Clinical Tree
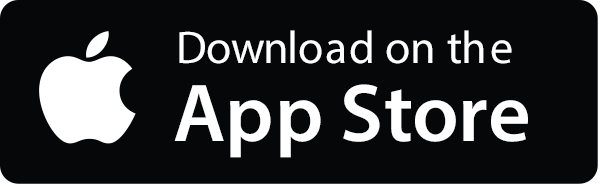
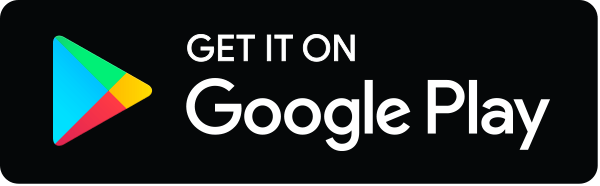