13 It’s Not Just the Tumor: Treatment Effects
13.1 Introduction
Imaging plays a major role in monitoring brain tumor patients; however, assessment of treatment response is currently limited to sequential measurements of only the contrast-enhancing component of the lesion using either Macdonald criteria or Response Evaluation Criteria in Solid Tumors (RECIST). 1 These methods use changes in the size of the contrast enhancement as a biomarker for treatment response or failure. However, because these methods rely on direct correlation between changes in enhancement and disease response or progression, they may not completely assess the nonenhancing component of the tumor. More importantly, these imaging biomarkers may not provide an accurate assessment of the tumor physiology, vascularity, and metabolism.
Brain tumor enhancement, or lack thereof, is affected by the presence and integrity of the blood–brain barrier (BBB). Therefore, any factors affecting the BBB will also affect the enhancement pattern on imaging. And, although changes in enhancement pattern can result from disease response or progression, a number of additional factors, particularly those related to the therapy, can affect enhancement patterns as well. For example, processes such as postictal changes, postoperative infarcts, or treatment-related inflammatory processes can disrupt the BBB, resulting in increased enhancement, thus mimicking disease progression and referred to as pseudoprogression. In addition, other factors, such as steroid treatment and antiangiogenic agents, can actually improve the integrity of the BBB, leading to decreased enhancement without much cytotoxic effect and hence mimicking treatment response, also referred to as pseudoresponse. 1 , 2 The Response Assessment in Neuro-Oncology (RANO) Working Group recently updated the criteria and included assessment of the nonenhancing part of the tumor based on T2 fluid-attenuated inversion recovery (T2/FLAIR) imaging to counter the issue of pseudoresponse following treatment with antiangiogenic agents. 3
Other common adverse treatment effects seen in busy neuro-oncology centers are related to radiation therapy, with delayed radiation necrosis being a common nemesis. Differentiating radiation necrosis from tumor progression is usually not possible based solely on morphological magnetic resonance imaging (MRI) features. 4
13.2 Clinical Implications
Accurately identifying treatment effects is especially challenging for brain tumors due to the rapidly changing and evolving field of combination therapy regimens, which is a consequence of the disease’s poor overall prognosis. In 2005 a study by Stupp et al found a small advantage in progression-free survival (PFS), as well as a significant benefit in 2-year overall survival with the use of temozolomide plus radiation therapy. 5 This has since become the standard of care following tumor resection. 6 In 2009 the U.S. Food and Drug Administration approved the use of bevacizumab, an antiangiogenic agent, for the treatment of recurrent gliomas. 7 Although new treatments offer hope for improving the overall dismal prognosis, they also lead to new imaging patterns, which can create confusion in differentiating treatment response or failure from treatment effects. Imaging biomarkers that provide early indication of treatment failure and timely opportunity to select an alternative treatment, or those that correctly diagnose treatment effects and prevent premature discontinuation of an effective treatment, are therefore of very active clinical interest. In particular, many studies rely on PFS and radiographic response (RR) to determine the effectiveness of a given therapy. However, because these methods have traditionally relied on changes in enhancement as the major differentiating factor, incorrectly categorizing these changes can lead to falsely high response or failure rates for a particular therapy. 8 Functional imaging modalities can provide additional information that may help differentiate treatment effects from true tumor progression and are discussed in this chapter.
13.3 Radiation Therapy–Induced Treatment Effects and Toxicity
Radiation therapy combined with chemotherapy is the standard of care following resection of high-grade gliomas. In patients undergoing treatment for malignant gliomas, radiation therapy is typically administered over a span of 6 to 7 weeks, usually consisting of fractionated focal irradiation at a dose of 2 Gy per fraction for a total dose of 60 Gy. 5 , 9 Although this therapy regimen has led to improved overall patient survival, delivering high doses of radiation can lead to a number of treatment-related effects, which can confound the overall clinical and imaging picture. Understanding the presentation of these changes from both a clinical and an imaging standpoint is, therefore, essential to ensure proper patient management.
The incidence and severity of radiation-induced effects depend on a number of factors, including patient factors, such as overall patient health; factors related to the treatment regimen, such as the use of concomitant chemotherapy; or factors related to the radiation therapy itself, such as the amount and type of radiation being administered. 10 In addition, recent studies have identified tumor-specific factors that also may influence the frequency of certain radiation-related effects. A study by Brandes et al in 2008 found that the incidence of pseudoprogression, a well-known cause of postradiation changes mimicking tumor progression, is significantly correlated with methylation of O 6 -methylguanine-DNA methyltransferase, a DNA repair enzyme, and in fact may be associated with survival benefit. 11
Classically, the effects of radiation on the central nervous system have been divided into three categories: acute, early delayed or subacute, and late effects. 12 , 13
Acute effects of radiation therapy occur either during or within days to weeks following therapy. 10 , 12 , 13 Symptoms, which are generally mild and typically reversible, can include fatigue, dizziness, and signs of elevated intracranial pressure. 12 , 13
Subacute or early delayed effects of radiation therapy generally occur a few weeks to several months following radiation treatment. 10 , 12 Symptoms during this period, which are also typically reversible, include generalized weakness and somnolence and are thought to relate to transient demyelination. 13 During this subacute phase of radiation injury the relatively new phenomenon known as pseudoprogression may arise. Pseudoprogression refers to a treatment-related increase in enhancing lesion size and/or edema without actual increase in tumor burden. This is confirmed on follow-up imaging demonstrating either regression or lack of progression of the imaging abnormalities without any change in therapy regimen (Fig. 13.1). Although a number of factors can lead to increased enhancement or edema following treatment, including postictal changes, postoperative infarcts, and changes in steroid dose, radiation injury has also been identified as a causative factor. Pseudoprogression is believed to occur more frequently in patients undergoing combination therapy with radiation and temozolomide, although it is also seen in patients treated only with radiation. 14 Pseudoprogression typically occurs in the 2 to 6 month period following chemoradiation, with a median occurrence time of 3 months. 15 The reported incidence of pseudoprogression ranges from 15 to 30%. 12 Pseudoprogression can have important implications in patient treatment. First, mischaracterization of recurrent tumor as pseudoprogression or vice versa can either delay necessary changes in therapy or result in continuation of an ineffective treatment. 3 Second, pseudoprogression may have important implications for patient prognosis and has been, in fact, associated with improved survival. 11
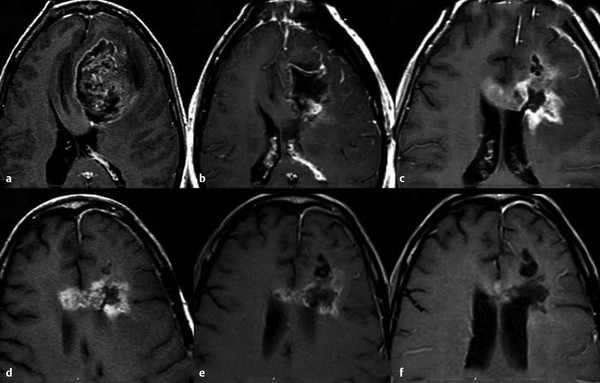
Late effects of radiation classically relate to delayed radiation necrosis. These effects generally occur between 3 and 12 months following radiation therapy, although they can be seen up to decades following completion of treatment. 12 , 16 Radiation necrosis is reported to occur in approximately 3 to 24% of adults undergoing standard radiation therapy. 14 Clinical symptoms related to these late effects of therapy, including focal neurological deficits, seizures, and cognitive dysfunction, as well as symptoms related to swelling, mass effect, and increased intracranial pressure, are typically more severe than those seen early on and are often irreversible. 13 , 17 In addition, because the clinical presentation of radiation necrosis is difficult to distinguish from tumor progression, decisions regarding patient management are often complicated.
13.3.1 Pathophysiology of Radiation-Induced Central Nervous System Injury
The exact pathophysiological mechanisms leading to radiation damage are still under investigation. However, understanding the changes occurring within the local tissues following radiation therapy is important when attempting to differentiate tumor recurrence from treatment effects based on imaging analysis. The ability of normal brain parenchyma to withstand radiation damage is dependent on a number of factors, including total dose administered, length of exposure, volume of tissue irradiated, as well as other concomitant therapies. 13 Although the pathophysiology leading to radiation-induced brain injury is likely a complex process involving a number of causes, two primary factors have classically been described: injury to the glial cells and injury to the endothelial cells of the cerebral vasculature. 13 , 18 , 19
Studies have shown that oligodendrocytes are radiosensitive, with cell death occurring soon after radiation exposure. 20 In addition, radiation leads to loss of O-2A progenitor cells, which are the source of new oligodendrocytes. Given the radiosensitivity of oligodendrocytes and their precursors, they have long been suspected as a potential etiology for radiation injury. Supporting this is the fact that oligodendrocytes are responsible for the production of myelin, and demyelination is one of the histological changes seen following early delayed radiation injury. 21 However, although radiation effects on oligodendrocytes and their precursors may explain the transient demyelination observed in the early stages of radiation injury, the time course of these changes does not explain the radiation necrosis seen in the later stages. 13 , 22
In addition to radiation’s effect on oligodendrocytes and their precursors, the early effects of radiation also result in damage and breakdown of the BBB, likely resulting from loosened endothelial tight junctions, vascular leakage, and endothelial cell death. 23 , 24 Although this initial effect on the BBB is transient, the endothelial cells have already suffered significant chromosomal damage that leads to declining cell numbers. 23 Eventually, once the number of functioning endothelial cells falls below a certain threshold, the integrity of the BBB is severely inhibited, leading to an increase in vascular permeability. 18 , 23 Such damage to the vasculature subsequently leads to thickening of the vessel walls and hyalinization, as well as fibrinoid necrosis with resultant thrombosis and infarction, finally leading to coagulative necrosis within the perivascular parenchyma. 25
In addition to the direct deleterious effects of radiation on the vasculature and glial cells, secondary injury due to production of reactive oxygen species and the production of other inflammatory mediators by increased numbers of reactive cells at the site of tissue injury have also been implicated as causes of late effects of radiation therapy. 13
Pseudoprogression
Although the exact mechanism for pseudoprogression is not known, it likely relates to the changes seen in the BBB during the early subacute phase of radiation injury. These changes result in a combination of increased inflammation, edema, and abnormal vessel permeability in the local tissues following treatment, which in turn allows the movement of fluid into the interstitial space, resulting in brain edema. 11 This alteration in capillary permeability, while enhancing the effect of chemotherapy by allowing maximized uptake of the drug, also leads to increased contrast enhancement. 11 This increased enhancement and edema results in an overall imaging appearance that mimics tumor progression while actually likely reflecting treatment effect, thus the name pseudoprogression.
13.3.2 Radiation Toxicity: Role of Imaging
Acute Radiation Injury
The typical imaging appearance of acute radiation injury on standard MRI is focal edema, which presents as increased T2/FLAIR signal abnormality. 12 A 2009 study by Cao et al found that both vascular volume and BBB permeability showed an initial increase during the course of radiation therapy followed by a gradual decrease after radiation therapy completion. 26 The study also found that areas of the brain receiving the highest doses of radiation showed the most rapid and significant changes. 26 The changes in vascular volume were felt to be secondary to vessel dilation in response to radiation with the increased BBB permeability resulting from endothelial cell death and apoptosis. 26 An earlier study by Cao et al also found that, although increased gadolinium diethylenetriamine penta-acetic acid (Gd-DTPA) uptake was observed in the nonenhancing tumor region following radiation therapy, a similar phenomenon was not seen in the remaining brain during the course of radiation therapy, suggesting a selective effect of radiation on the blood–tumor barrier over the BBB. 24
Subacute Radiation Injury (Pseudoprogression)
Unfortunately, the morphological features identified in pseudoprogression, including increased enhancement and/or edema, are similar to those seen with early tumor progression. As a result, differentiating the two using morphological MRI is not possible. A study by Young et al assessing the use of conventional MRI in diagnosing pseudoprogression found it to be of limited utility. 27 However, although the study failed to find a sign with sufficient negative predictive value to confidently diagnose pseudoprogression, it did find that subependymal spread of an enhancing lesion was a useful marker for early progression. 27 Similarly, a recent study by Agarwal et al evaluating 20 morphological features in an attempt to differentiate pseudoprogression from early tumor progression found that the only statistically significant findings were larger size of the T2/FLAIR signal abnormality and larger size of the enhancing component, both of which favored early tumor progression. 28 Although the presence of ependymal enhancement, poorly defined margin of the enhancing component, and corpus callosum involvement were all found more often in early tumor progression, the findings were not statistically significant. 28 Despite these findings, currently the only method to definitively diagnose pseudoprogression is based retrospectively on serial follow-up imaging.
Functional Imaging Techniques: Perfusion Imaging
Contrast enhancement in pseudoprogression, a result of increased vessel permeability and disruption of the BBB, is indistinguishable on MRI from that produced by the process of angiogenesis in progressive tumor, thus precluding their differentiation. However, by assessing changes occurring on the microvasculature level, perfusion imaging allows quantification of certain parameters, including tumor blood volume and vascular permeability, thus providing a potential tool for discriminating between the two processes. Whereas many studies have assessed the performance of perfusion imaging in differentiating radiation necrosis and recurrent progressive tumor, fewer have assessed pseudoprogression. However, radiation necrosis and pseudo-progression have different pathophysiologic mechanisms, but they share a number of similarities, resulting in similar imaging features. 29 In particular, enhancing lesions related to pseudoprogression should demonstrate lower blood volume and permeability when compared to recurrent tumor (Fig. 13.2), reflecting the absence of angiogenesis, as well as lower-grade leakiness due to radiation-induced BBB disruption rather than the marked leakiness associated with angiogenesis and tumor vasculature in recurrent tumor. 29
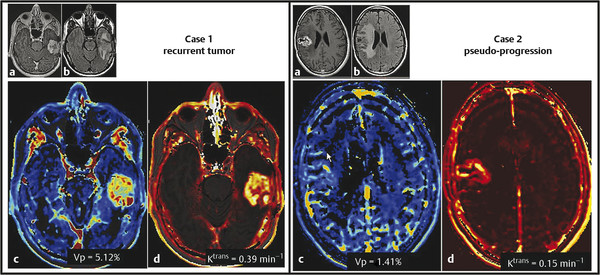
A study by Mangla et al confirmed these findings, demonstrating that relative cerebral blood volume (rCBV) at 1 month was able to distinguish pseudoprogression from recurrent progressive disease with a sensitivity of 77% and specificity of 86%. 30 Similarly, a study by Young et al found that pseudoprogression demonstrated a lower median rCBV and lower permeability (measured by percent signal recovery) when compared to disease progression. 29 Gahramanov et al also found lower rCBV in cases of pseudoprogression and, in addition, found that ferumoxytol, a blood pool agent, offered a potentially simpler model for blood volume assessment by not requiring correction for leakage of contrast. 31
Given the complicated multicompartment physiological models required to quantitatively assess tumor blood volume and permeability, which potentially limit its clinical applicability, model-free “semiquantitative” indices have also been used in the past to assess tissue perfusion. 32 These semiquantitative methods, in addition to assessing the shape of the uptake and washout of the contrast agent, also offer a variety of non-model-based semiquantitative indices, which provide a more objective means of assessment. These indices include maximum slope of enhancement in the initial vascular phase (MSIVP), which assesses change of signal intensity per second; normalized slope of the delayed equilibrium phase (nSDEP), which is the slope of the fitted linear curve to the final 25% of samples; as well as the initial area under the time–intensity curve (IAUC) at 60 and 120 seconds (IAUC60 and IAUC120). 32 Using these semiquantitative indices, Jain et al found that pseudoprogression demonstrated a lower mean MSIVP, lower nIAUC60, and higher nSDEP compared to early tumor progression (Fig. 13.3). 32
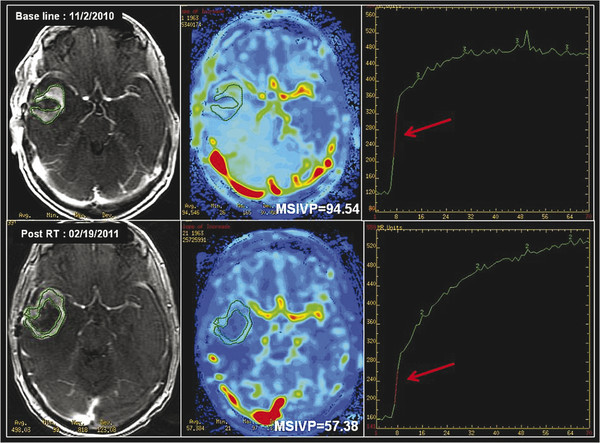
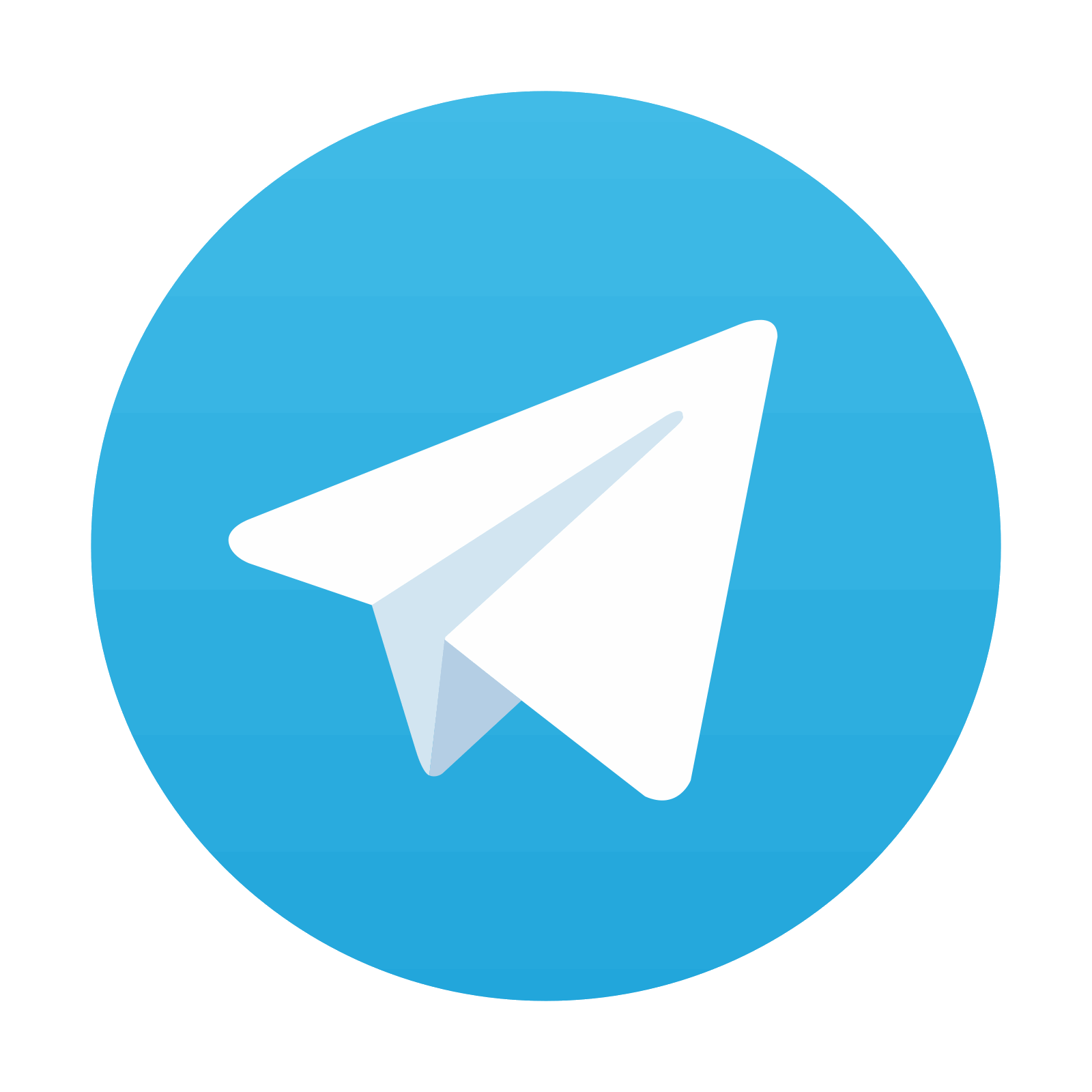
Stay updated, free articles. Join our Telegram channel
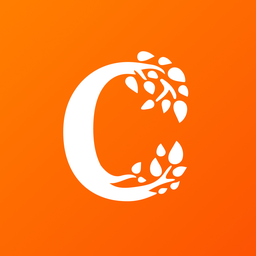
Full access? Get Clinical Tree
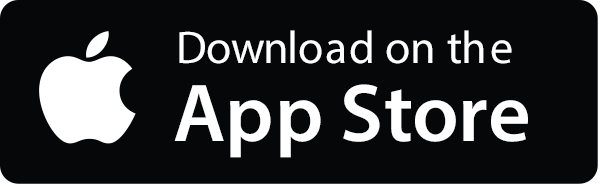
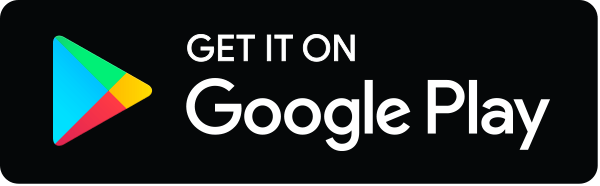
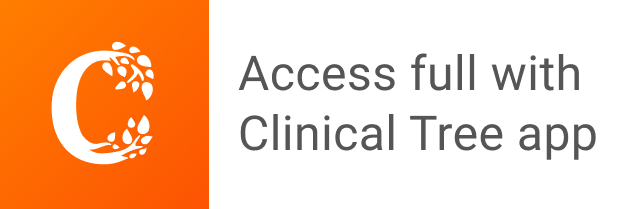