- Basic components of a magnetic resonance (MR) scanner and the types of magnetic fields each component produces
- Potential biological effects of static magnetic fields and field gradients during interactions with magnetic objects
- Interaction of radiofrequency (RF) magnetic fields with normal tissue and electrically conducting materials
- Biological effects of time-varying magnetic field gradients
- Potential impacts of magnetic resonance imaging (MRI) on subjects with implanted medical devices
Introduction
Regarding biological effects, the first thing to note about magnetic resonance imaging (MRI) is the lack of ionizing radiation. During a normal magnetic resonance (MR) scan, the patient’s body absorbs energy; however, the absorbed energy is in the radiofrequency (RF) portion of the electromagnetic spectrum. As explained in Chapter 3, RF electromagnetic energy, when absorbed by body tissues, causes heating but not ionization. In addition to mild heating, there are other biological effects that may result during MRI. Some of these are routinely encountered (such as audible noise); others occur very infrequently (such as RF burns). Although extremely rare, improper MR procedures have resulted in serious injury or death. In general, MRI is safe and imparts absolutely no ionizing radiation dose.
In order to appreciate the range of potential biological effects of MRI, it is necessary to first become familiar with the basic components of an MR scanner, and the purpose and type of magnetic field produced by each component.
Basic Principles of MRI
The ability to noninvasively image the body by MRI is based on the manipulation and detection of the magnetic properties of hydrogen nuclei. Hydrogen is the most abundant element in the body, and the hydrogen nucleus has a magnetic moment, with magnetic behavior similar to that of the electron or a compass needle. The electron is a “spin ½” particle, meaning that it is observed in one of two states, referred to as “up” and “down.” Similarly, the spin of the hydrogen nucleus (i.e., proton) assumes either an “up” or “down” orientation with respect to an externally applied magnetic field. Note that electron spins are what create magnetism in magnetic materials, such as iron. The hydrogen magnetic moment, however, is about 2000 times weaker than that of the electron, and therefore hydrogenous materials (e.g., plastic, water, and tissue) do not stick to magnets. Fortunately, the very weak magnetism of hydrogen nuclei is adequate to enable MRI.
MRI involves first aligning the hydrogen spins using a strong external magnetic field, and then perturbing the spin direction in a way that produces a voltage in a detection coil. The polarizing field is typically provided by a large donut-shaped superconducting magnet that produces a strong, constant magnetic field in the center of the donut. Figure 17.1a is a photograph of a typical clinical MR scanner, and Fig. 17.1b shows a cutaway indicating the location of the various hardware components discussed in this chapter.
Figure 17.1 (a) Photograph of a clinical MR scanner. (b) Cutaway diagram showing the essential components of an MR scanner, including the main magnet, RF coil, and gradient coils.
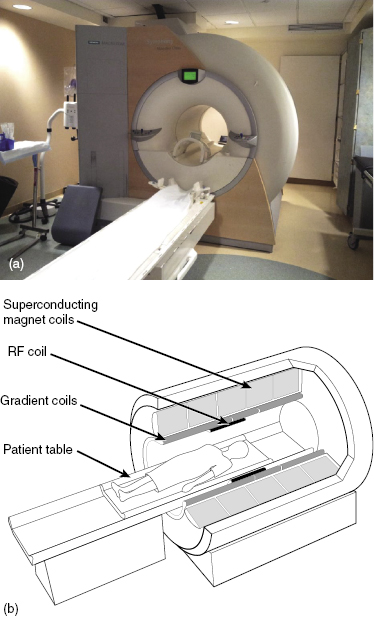
The perturbation of the hydrogen spins and subsequent voltage detection are performed by the much smaller RF coil, which basically acts as a two-way radio antenna that transmits and receives RF signals. (In modern MRI systems, the transmit and receive functions may be performed by separate circuit elements, or there may be multiple parallel receive circuit elements, but the ensemble of RF circuits is still referred to as “the coil.”) The RF coil is wrapped around (or placed against) the part of the body that is being imaged.
Additionally, during the MRI acquisition, another set of coils (the “imaging gradient coils” or “gradients”) surrounding the patient are pulsed on and off in a way that encodes information about spatial position in the voltage signals detected from the hydrogen spins. The encoded spatial information in the voltage signals is decoded using computer algorithms (e.g., fast Fourier transform) to create a two-dimensional or three-dimensional image. Because the MR signals generated from hydrogen in different tissue types differ in magnitude and duration, the differing signal intensities from different tissues (e.g., muscle and fat) enable anatomic structures to be distinguished.
To summarize, MRI utilizes three main hardware components—(1) the main magnet (to align the spins), (2) the RF coil (to perturb the spins and detect voltage signals), and (3) the gradient coil set (to encode spatial information into the voltage signals). Each hardware component generates magnetic fields with different characteristics that result in different biological effects, as discussed in the following section.
Biological Effects of MRI
Main Magnet: Static Magnetic Field and Field Gradient Effects
The main magnet creates a magnetic field that is extremely strong, >1 tesla (T), spatially uniform across the patient’s body, and constant over time. The most common field strengths for clinical whole body magnets are 1.5 and 3 T. Whole body magnets with field strengths of 7 T or greater are used in research, but are not yet clinically approved. For comparison, the Earth’s magnetic field is about 0.00005 T = 0.5 gauss (G) (1 T = 10,000 G).
Whole body MRI magnets are generally superconducting solenoids. A solenoid is a coiled current-carrying wire (see Fig. 17.2a); the field inside of a cylindrical solenoid is uniform and parallel to the axis of the coil, as depicted by the gray magnetic field lines in Fig. 17.2b. A superconducting solenoid is immersed in liquid helium (at a temperature of −269°C) to maintain the superconducting state. Superconductivity (the flow of electrical current without resistance) is what maintains the magnetic field indefinitely without a constant input of electrical energy. In other words, the magnet is not “plugged in” during normal operation. Once the solenoid is magnetized, the magnetic field is maintained at a constant value over time, ideally for the entire life of the scanner (hence the term “static” field). As in computed tomography (CT), the patient is imaged in the center of the donut, but the length of the cylinder is much greater in an MR scanner than in a CT scanner. Thus, a common biological effect of MR is claustrophobia, which is occasionally serious enough to contraindicate MRI for some patients.
Figure 17.2 (a) A current-carrying wire, wrapped to form a solenoid, produces a magnetic field. (b) The gray lines represent the magnetic field of a solenoid, while the black dots represent the current-carrying wires (in cross section).
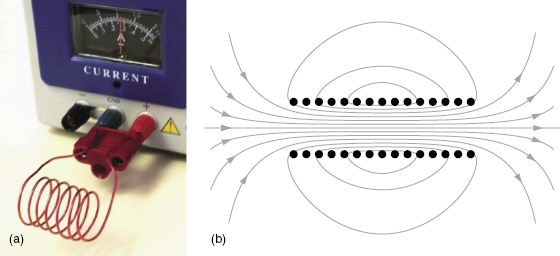
Brief exposures to static magnetic fields are not known to have any significant biological effects on mammals. This makes sense because mammals do not naturally have ferromagnetic materials in their bodies that would interact with static fields. Normal tissue does not interact with static fields, and therefore, tissue is essentially transparent to static fields (i.e., there is no attenuation). Ferromagnetic materials (so called because they typically contain iron) interact strongly with magnetic fields due to cooperative effects of the electron spins in the material. However, not all iron-containing substances are ferromagnetic. For example, the iron-containing hemoglobin in your blood exhibits very weak magnetism (it does not participate in cooperative magnetic effects) and is not significantly affected by MRI magnetic fields. Generally, the strong static magnetic field created by the main magnet only causes significant biological effects by interacting with foreign ferromagnetic objects inside or near the patient.
Torque
Just as the Earth’s magnetic field will orient a compass needle, the strong static field in an MR scanner will exert a torque (twisting force) on elongated magnetic objects to orient the long axis of the object parallel to the magnetic field. Because the torque is proportional to the strength of the magnetic field, the torque generated by an MR magnet is tens of thousands of times stronger than the torque caused by the Earth’s field. For example, if a child has swallowed a straight pin, the child should not undergo MRI, because the pin would rapidly rotate to align with the main magnetic field. For this reason, people with a known history of injury involving penetrating metallic objects may be screened using X-ray imaging prior to undergoing MRI.
Translational Force
The translational magnetic force (the force that causes a magnetic object to move toward a magnet) is proportional to the spatial gradient of the magnetic field. A spatial gradient is a change in the magnetic field strength as a function of position. Note that a perfectly spatially uniform magnetic field does not cause any translational force, even if the field is extremely strong. However, the field of an MRI magnet is only spatially uniform in the center of the bore. In Fig. 17.2b, the magnetic field lines from a solenoid are shown. The gray lines represent the magnetic field, and the black dots represent the turns of wire forming the solenoid (in cross section). The magnetic field is strong (denoted by the density of the lines) and uniform (denoted by parallel lines) inside the solenoid, but the field becomes rapidly weaker (lines are less dense) and nonuniform (lines are not parallel) as one moves outside of the solenoid. In general, the field strength of any magnet, including MRI magnets, decreases as one moves further away from the magnet. Magnetic forces (e.g., the pull of a refrigerator magnet) are caused by the spatial variation of the magnetic field near the magnet.
Projectile Effect
A rare but significant biological effect of the main magnetic field occurs when someone accidentally brings a ferromagnetic object (e.g., a pair of scissors or an oxygen bottle) into the MRI suite. The object can become a dangerous projectile due to the strong attraction of the object to the “mouth” of the magnet bore, the location where the field strength is changing rapidly with distance (i.e., where the gradient is the strongest). Note the position of the gas cylinder in Fig. 17.3, which shows the result of bringing a non-MR-safe object into an MRI suite. If the ferromagnetic object is either sharp or massive, this phenomenon can result in serious injury, or even death, to patients or others in the MR suite. Note that the serious biological effect is caused by the impact with the projectile and is not a direct effect of the magnetic field on the subject.
Figure 17.3 A non-MR-safe oxygen bottle, stuck in the bore of an MR scanner at the position where the field gradient is the strongest. Careful screening of everything entering the MR scanner room is necessary to prevent serious accidents.
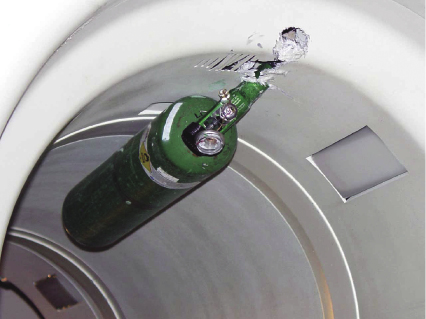
How do projectile accidents happen? Keep in mind that the magnetic field decreases as a function of distance (d) with a 1/d3 dependence, and the spatial gradient decreases with a 1/d4 dependence. This very strong dependence of the gradient strength on distance means that the magnetic force on an object can be nearly imperceptible at one point in the room (perhaps a meter or so from the main magnet), but the force becomes extremely strong if the object is moved just a few centimeters closer. Thus, the danger with objects becoming projectiles is related to how suddenly the force changes from insignificant to dangerous. If a person is walking toward the magnet carrying a magnetic object, the time between first feeling the force and the object clanging uncontrollably into the mouth of the magnet is typically less than the person’s reaction time—by the time a problem is perceived, the force is too great to overcome.
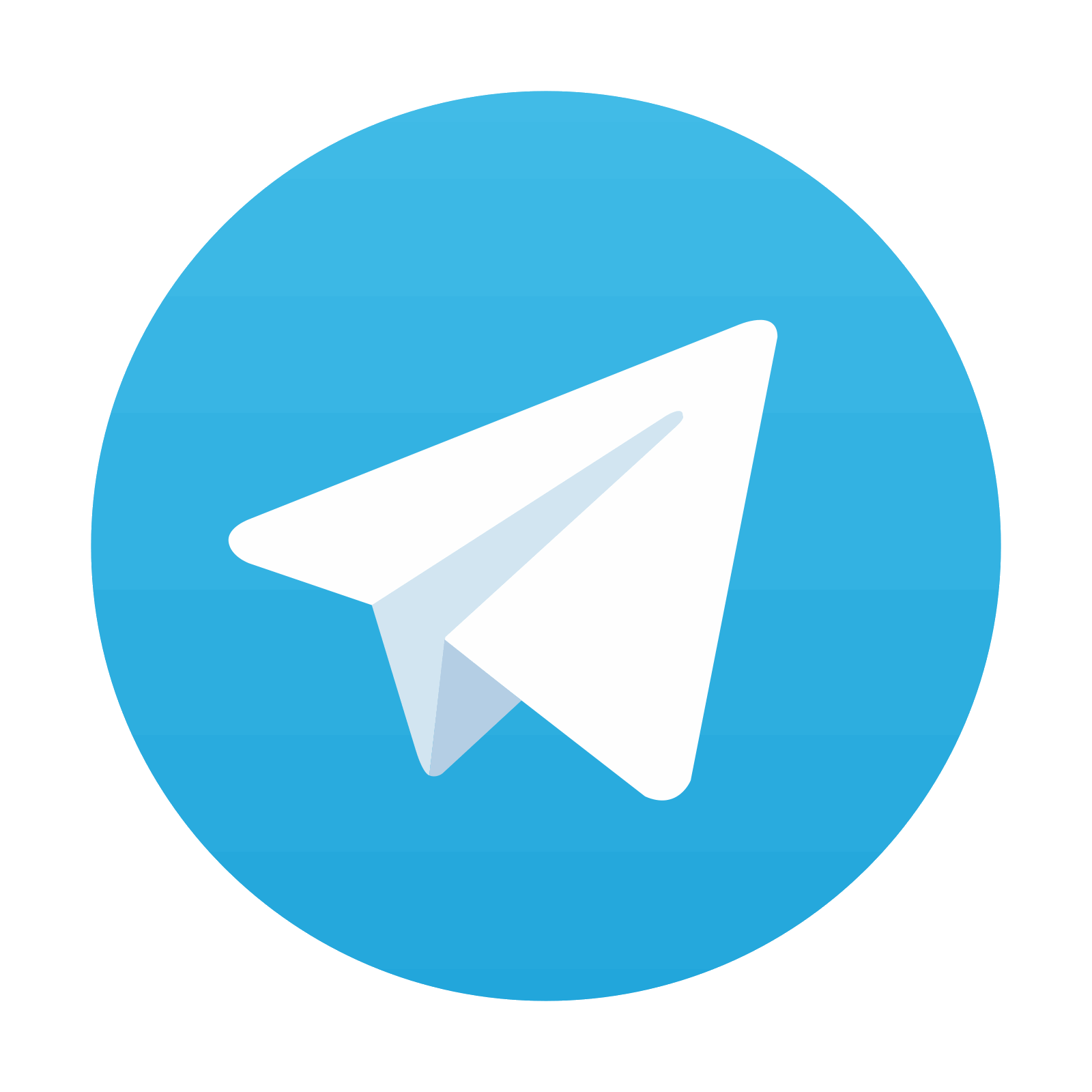
Stay updated, free articles. Join our Telegram channel
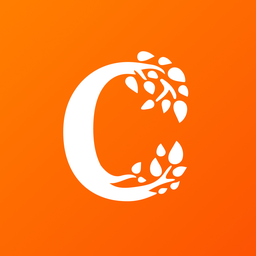
Full access? Get Clinical Tree
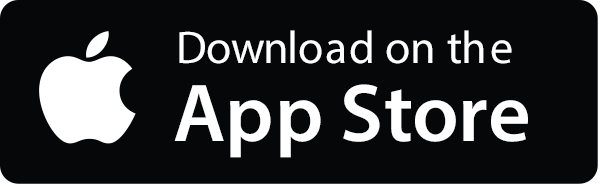
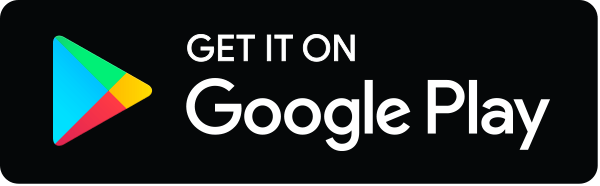